Accessibility Links
- Skip to content
- Skip to search IOPscience
- Skip to Journals list
- Accessibility help
- Accessibility Help
Click here to close this panel.
Purpose-led Publishing is a coalition of three not-for-profit publishers in the field of physical sciences: AIP Publishing, the American Physical Society and IOP Publishing.
Together, as publishers that will always put purpose above profit, we have defined a set of industry standards that underpin high-quality, ethical scholarly communications.
We are proudly declaring that science is our only shareholder.
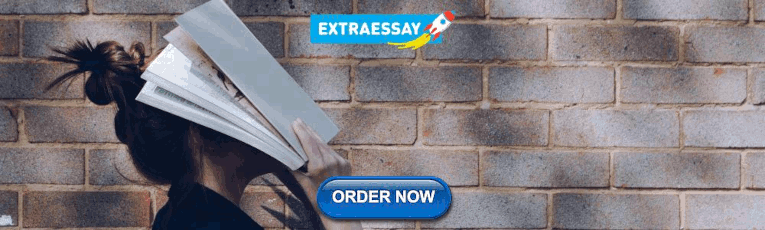
The rise of electric vehicles—2020 status and future expectations
Matteo Muratori 13,1 , Marcus Alexander 2 , Doug Arent 1 , Morgan Bazilian 3 , Pierpaolo Cazzola 4 , Ercan M Dede 5 , John Farrell 1 , Chris Gearhart 1 , David Greene 6 , Alan Jenn 7 , Matthew Keyser 1 , Timothy Lipman 8 , Sreekant Narumanchi 1 , Ahmad Pesaran 1 , Ramteen Sioshansi 9 , Emilia Suomalainen 10 , Gil Tal 7 , Kevin Walkowicz 11 and Jacob Ward 12
Published 25 March 2021 • © 2021 IOP Publishing Ltd Progress in Energy , Volume 3 , Number 2 Focus on Transport Electrification Citation Matteo Muratori et al 2021 Prog. Energy 3 022002 DOI 10.1088/2516-1083/abe0ad
Article metrics
70417 Total downloads
Permissions
Get permission to re-use this article
Share this article
Author e-mails.
Author affiliations
1 National Renewable Energy Laboratory, Golden, CO, United States of America
2 Electric Power Research Institute, Palo Alto, CA, United States of America
3 Colorado School of Mines, Golden, CO, United States of America
4 International Transport Forum in Paris, France
5 Toyota Research Institute of North America, Ann Arbour, MI, United States of America
6 University of Tennessee, Knoxville, TN, United States of America
7 University of California, Davis, CA, United States of America
8 University of California, Berkeley, CA, United States of America
9 The Ohio State University, Columbus, OH, United States of America
10 Institut VEDECOM, Versailles, France
11 Calstart, Pasadena, CA, United States of America
12 Carnegie Mellon University, Pittsburgh, PA, United States of America
Author notes
13 Author to whom any correspondence should be addressed.
Matteo Muratori https://orcid.org/0000-0003-1688-6742
Doug Arent https://orcid.org/0000-0002-4219-3950
Morgan Bazilian https://orcid.org/0000-0003-1650-8071
Ahmad Pesaran https://orcid.org/0000-0003-0666-1021
Emilia Suomalainen https://orcid.org/0000-0002-6339-2932
Gil Tal https://orcid.org/0000-0001-7843-3664
Jacob Ward https://orcid.org/0000-0002-8278-8940
- Received 3 August 2020
- Accepted 27 January 2021
- Published 25 March 2021
Peer review information
Method : Single-anonymous Revisions: 3 Screened for originality? Yes
Buy this article in print
Electric vehicles (EVs) are experiencing a rise in popularity over the past few years as the technology has matured and costs have declined, and support for clean transportation has promoted awareness, increased charging opportunities, and facilitated EV adoption. Suitably, a vast body of literature has been produced exploring various facets of EVs and their role in transportation and energy systems. This paper provides a timely and comprehensive review of scientific studies looking at various aspects of EVs, including: (a) an overview of the status of the light-duty-EV market and current projections for future adoption; (b) insights on market opportunities beyond light-duty EVs; (c) a review of cost and performance evolution for batteries, power electronics, and electric machines that are key components of EV success; (d) charging-infrastructure status with a focus on modeling and studies that are used to project charging-infrastructure requirements and the economics of public charging; (e) an overview of the impact of EV charging on power systems at multiple scales, ranging from bulk power systems to distribution networks; (f) insights into life-cycle cost and emissions studies focusing on EVs; and (g) future expectations and synergies between EVs and other emerging trends and technologies. The goal of this paper is to provide readers with a snapshot of the current state of the art and help navigate this vast literature by comparing studies critically and comprehensively and synthesizing general insights. This detailed review paints a positive picture for the future of EVs for on-road transportation, and the authors remain hopeful that remaining technology, regulatory, societal, behavioral, and business-model barriers can be addressed over time to support a transition toward cleaner, more efficient, and affordable transportation solutions for all.
Export citation and abstract BibTeX RIS
This article was updated on 29 April 2021 to add the name of the fifth author and to correct the name of the eighth author.
1. Introduction
First introduced at the end of the 1800s, electric vehicles (EVs) 12 have been experiencing a rise in popularity over the past few years as the technology has matured and costs (especially of batteries) have declined substantially. Worldwide support for clean transportation options (i.e. low emissions of greenhouse gasses [GHG] to mitigate climate change and criteria pollutants) has promoted awareness, increased charging opportunities, and facilitated adoption of EVs. EVs present numerous advantages compared to fossil-fueled internal-combustion-engine vehicles (ICEVs), inter alia: zero tailpipe emissions, no reliance on petroleum, improved fuel economy, lower maintenance, and improved driving experience (e.g. acceleration, noise reduction, and convenient home and opportunity recharging). Further, when charged with clean electricity, EVs provide a viable pathway to reduce overall GHG emissions and decarbonize on-road transportation. This decarbonization potential is important, given limited alternative options to liquid fossil fuels. The ability of EVs to reduce GHG emissions is dependent, however, upon clean electricity. Therefore, EV success is intertwined closely with the prospect of abundant and affordable renewable electricity (in particular solar and wind electricity) that is poised to transform power systems (Jacobson et al 2015 , Kroposki et al 2017 , Gielen et al 2019 , IEA 2020b ). Coordinated actions can produce beneficial synergies between EVs and power systems and support renewable-energy integration to optimize energy systems of the future to benefit users and offer decarbonization across sectors (CEM 2020 ). A cross-sectoral approach across the entire energy system is required to realise clean future transformation pathways (Hansen et al 2019 ). EVs are expected to play a critical role in the power system of the future (Muratori and Mai).
EV success is increasing rapidly since the mid-2010s. EV sales are breaking previous records every year, especially for light-duty vehicles (LDVs), buses, and smaller vehicles such as three-wheelers, mopeds, kick-scooters, and e-bikes (IEA 2017 , 2018a , 2019 , 2020 ). To date, global automakers are committing more than $140 billion to transportation electrification, and 50 light-duty EV models are available commercially in the U.S. market (Moore and Bullard 2020 ). Approximately 130 EV models are anticipated by 2023 (AFDC 2020 , Moore and Bullard 2020 ). Future projections of the role of EVs in LDV markets vary widely, with estimates ranging from limited success (∼10% of sales in 2050) to full market dominance, with EVs accounting for 100% of LDV sales well before 2050. Many studies project that EVs will become economically competitive with ICEVs in the near future or that they are already cost-competitive for some applications (Weldon et al 2018 , Sioshansi and Webb 2019 , Yale E360 2019 , Kapustin and Grushevenko 2020 ). However, widespread adoption requires more than economic competitiveness, especially for personally owned vehicles. Behavioral and non-financial preferences of individuals on different technologies and mobility options are also important (Lavieri et al 2017 , Li et al 2017 , McCollum et al 2018 , Ramea et al 2018 ). EV adoption beyond LDVs has been focused on buses, with significant adoption in several regions (especially China). Electric trucks also are receiving great attention, and Bloomberg New Energy Finance (BloombergNEF) projects that by 2025, alternative fuels will compete with, or outcompete, diesel in long-haul trucking applications (Moore and Bullard 2020 ). These recent successes are being driven by technological progress, especially in batteries and power electronics, greater availability of charging infrastructure, policy support driven by environmental benefits, and consumer acceptance. EV adoption is engendering a virtuous circle of technology improvements and cost reductions that is enabled and constrained by positive feedbacks arising from scale and learning by doing, research and development, charging-infrastructure coverage and utilization, and consumer experience and familiarity with EVs.
Vehicle electrification is a game-changer for the transportation sector due to major energy and environmental implications driven by high vehicle efficiency (EVs are approximately 3–4 times more efficient than comparable ICEVs), zero tailpipe emissions, and reduced petroleum dependency (great fuel diversity and flexibility exist in electricity production). Far-reaching implications for vehicle-grid integration extend to the electricity sector and to the broader energy system. A revealing example of the role of EVs in broader energy-transformation scenarios is provided by Muratori and Mai, who summarize results from 159 scenarios underpinning the special report on Global Warming of 1.5 °C (SR1.5) by Intergovernmental Panel on Climate Change (IPCC). Muratori and Mai also show that transportation represents only ∼2% of global electricity demand currently (with rail responsible for more than two-thirds of this total). They show that electricity is projected to provide 18% of all transportation-energy needs by 2050 for the median IPCC scenario, which would account for 10% of total electricity demand. Most of this electricity use is targeted toward on-road vehicle electrification. These projections are the result of modeling and simulations that are struggling to keep pace with the EV revolution and its role in energy-transformation scenarios as EV technologies and mobility are evolving rapidly (McCollum et al 2017 , Venturini et al 2019 , Muratori et al 2020 ). Recent studies explore higher transportation-electrification scenarios: for example, Mai et al ( 2018 ) report a scenario in which 75% of on-road miles are powered by electricity, and transportation represents almost a quarter of total electricity use during 2050.
Vehicle electrification is a disruptive element in energy-system evolution that radically changes the roles of different sectors, technologies, and fuels in long-term transformation scenarios. Traditionally, energy-system-transformation studies project minimal end-use changes in transportation-energy use over time (limited mode shifting and adoption of alternative fuels), and the sector is portrayed as a 'roadblock' to decarbonization. In many decarbonization scenarios, transportation is seen traditionally as one of the biggest hurdles to achieve emissions reductions (The White House 2016 ). These scenarios rely on greater changes in the energy supply to reduce emissions and petroleum dependency (e.g. large-scale use of bioenergy, often coupled to carbon capture and sequestration) rather than demand-side transformations (IPCC 2014 , Pietzcker et al 2014 , Creutzig et al 2015 , Muratori et al 2017 , Santos 2017 ). In most of these studies, electrification is limited to some transportation modes (e.g. light-duty), and EVs are not expected to replace ICEVs fully (The White House 2016 ). More recently, however, major mobility disruptions (e.g. use of ride-hailing and vehicle ride-sharing) and massive EV adoption across multiple applications are proposed (Edelenbosch et al 2017 , Van Vuuren et al 2017 , Hill et al 2019 , E3 2020 , Zhang and Fujimori 2020 ). These mobility disruptions allow for more radical changes and increase the decarbonization role of transportation and highlight the integration opportunities between transportation and energy supply, especially within the electricity sector. For example, Zhang and Fujimori ( 2020 ) highlight that for vehicle electrification to contribute to climate-change mitigation, electricity generation needs to transition to clean sources. They note that EVs can reduce mitigation costs, implying a positive impact of transport policies on the economic system (Zhang and Fujimori 2020 ). In-line with these projections, many countries are establishing increasingly stringent and ambitious targets to support transport electrification and in some cases ban conventional fossil fuel vehicles (Wentland 2016 , Dhar et al 2017 , Coren 2018 , CARB 2020 , State of California 2020 ).
EV charging undoubtedly will impact the electricity sector in terms of overall energy use, demand profiles, and synergies with electricity supply. Mai et al ( 2018 ) show that in a high-electrification scenario, transportation might grow from the current 0.2% to 23% of total U.S. electricity demand in 2050 and significantly impact system peak load and related capacity costs if not controlled properly. Widespread vehicle electrification will impact the electricity system across the board, including generation, transmission, and distribution. However, expected changes in U.S. electricity demand as a result of vehicle electrification are not greater than historical growth in load and peak demand. This finding suggests that bulk-generation capacity is expected to be available to support a growing EV fleet as it evolves over time, even with high EV-market growth (U.S. DRIVE 2019 ). At the same time, many studies have shown that 'smart charging' and vehicle-to-grid (V2G) services create opportunities to reduce system costs and facilitate the integration of variable renewable energy (VRE). Charging infrastructure that enables smart charging and alignment with VRE generation, as well as business models and programs to compensate EV owners for providing charging flexibility, are the most pressing required elements for successfully integrating EVs with bulk power systems. At the local level, EV charging could increase and change electricity loads significantly, which could impact distribution networks and power quality and reliability (FleetCarma 2019 ). Distribution-network impacts can be particularly critical for high-power charging and in cases in which many EVs are concentrated in a specific location, such as clusters of residential LDV charging and possibly fleet depots for commercial vehicles (Muratori 2018 ).
This paper provides a timely status of the literature on several aspects of EV markets, technologies, and future projections. The paper focuses on multiple facets that characterize technology status and the role of EVs in transportation decarbonization and broader energy-transformation pathways focusing on the U.S. context. As appropriate, global context is provided as well. Hybrid EVs (for which liquid fuel is the only source of energy) and fuel cell EVs (that power an electric powertrain with a fuel cell and on-board hydrogen storage) have some similarities with EVs and could complement them for many applications. However, these technologies are not reviewed in detail here. The remainder of this paper is structured as follows. Section 2 focuses on the status of the light-duty-EV market and provides a comparison of projections for future adoption. Section 3 provides insights on market opportunities beyond LDVs. Section 4 offers a review of cost and performance evolution for batteries, power electronics, and electric machines that are key components of EV success. Section 5 reviews charging-infrastructure status and focuses on modeling and analysis studies used to project charging-infrastructure requirements, the economics of public charging, and some considerations on cybersecurity and future technologies (e.g. wireless charging). Section 6 provides an overview of the impact of EV charging on power systems at multiple scales, ranging from bulk power systems to distribution networks. Section 7 provides insights into life-cycle cost and emissions studies focusing on EVs. Finally, section 8 touches on future expectations.
1.1. Summary of take-away points
1.1.1. ev adoption.
- The global rate of adoption of light-duty EVs (passenger cars) has increased rapidly since the mid-2010s, supported by three key pillars: improvements in battery technologies; a wide range of supportive policies to reduce emissions; and regulations and standards to promote energy efficiency and reduce petroleum consumption.
- Adoption of advanced technologies has been underestimated historically in modeling and analyses; EV adoption is projected to remain limited until 2030, and high uncertainty is shown afterward with widely different projections from different sources. However, EVs hold great promise to replace conventional LDVs affordably.
- Barriers to EV adoption to date include consumer skepticism toward new technology, high purchase prices, limited range and lack of charging infrastructure, and lack of available models and other supply constraints.
- A major challenge facing both manufacturers and end-users of medium- and heavy-duty EVs is the diverse set of operational requirements and duty cycles that the vehicles encounter in real-world operation.
- EVs appear to be well suited for short-haul trucking applications such as regional and local deliveries. The potential for battery-electric models to work well in long-haul on-road applications has yet to be established, with different studies indicating different opportunities.
1.1.2. Batteries and other EV technologies
- Over the last 10 years, the price of lithium-ion battery packs has dropped by more than 80% (from over $1000 kWh −1 to $156 kWh −1 at the end of 2019, BloombergNEF 2020 ). Further price reduction is needed to achieve EV purchase-price parity with ICEVs.
- Over the last 10 years, the specific energy of a lithium-ion battery cell has almost doubled, reaching 240 Wh kg −1 (BloombergNEF 2020 ), reducing battery weight significantly.
- Reducing or eliminating cobalt in lithium-ion batteries is an opportunity to lower costs and reduce reliance on a rare material with controversial supply chains.
- While batteries are playing a key role in the rise of EVs, power electronics and electric motors are also key components of an EV powertrain. Recent trends toward integration promise to deliver benefits in terms of increased power density, lower losses, and lower costs.
1.1.3. Charging infrastructure
- With a few million light-duty EVs on the road, currently, there is about one public charge point per ten battery electric vehicles (BEVs) in U.S. (although most vehicles have access to a residential charger).
- Given the importance of home charging (and the added convenience compared to traditional refueling at public stations), charging solutions in residential areas comprising attached or multi-unit dwellings is likely to be essential for EVs to be adopted at large scale.
- Although public charging infrastructure is clearly important to EV purchasers, how best to deploy charging infrastructure in terms of numbers, types, locations, and timing remains an active area for research.
- The economics of public charging vary with location and station configuration and depend critically on equipment and installation costs, incentives, non-fuel revenues, and retail electricity prices, which are heavily dependent on station utilization.
- The electrification of medium- and heavy-duty commercial trucks and buses might introduce unique charging and infrastructure requirements compared to those of light-duty passenger vehicles.
- Wireless charging, specifically high-power wireless charging (beyond level-2 power), could play a key role in providing an automated charging solution for tomorrow's automated vehicles.
1.1.4. Power system integration
- Accommodating EV charging at the bulk power-system level (generation and transmission) is different in each region, but there are no major known technical challenges or risks to support a growing EV fleet, especially in the near term (approximately one decade).
- At the local level, however, EV charging can increase and change electricity loads significantly, causing possible negative impacts on distribution networks, especially for high-power charging.
- The integration of EVs into power systems presents opportunities for synergistic improvement of the efficiency and economics of electromobility and electric power systems, and EVs can support grid planning and operations in several ways.
- There are still many challenges for effective EV-grid integration at large scale, linked not only to the technical aspects of vehicle-grid-integration (VGI) technology but also to societal, economic, business model, security, and regulatory aspects.
- VGI offers many opportunities that justify the efforts required to overcome these challenges. In addition to its services to the power system, VGI offers interesting perspectives for the full exploitation of synergies between EVs and VRE as both technologies promise large-scale deployment in the future.
1.1.5. Life-cycle cost and emissions
- Many factors contribute to variability in EV life-cycle emissions, mostly the carbon intensity of electricity, charging patterns, vehicle characteristics, and even local climate. Grid decarbonization is a prerequisite for EVs to provide major GHG-emissions reductions.
- Existing literature suggests that future EVs can offer 70%–90% lower GHG emissions compared to today's ICEVs, most obviously due to broad expectations for continued grid decarbonization.
- Operational costs of EVs (fuel and maintenance) are typically lower than those of ICEVs, largely because EVs are more efficient than ICEVs and have fewer moving parts.
1.1.6. Synergies with other technologies and future expectations
- Vehicle electrification fits in broader electrification and mobility macro-trends, including micro-mobility in urban areas, new mobility business models regarding ride-hailing and car-sharing, and automation that complement well with EVs.
- While EVs are a relatively new technology and automated vehicles are not readily available to the general public, the implications and potential synergies of these technologies operating in conjunction are significant.
- The coronavirus pandemic is impacting transportation markets negatively, including those for EVs, but long-term prospects remain undiminished.
- Several studies project major roles for EVs in the future, which is reflected in massive investment in vehicle development and commercialization, charging infrastructure, and further technology improvement. Consumer adoption and acceptance and technology progress form a virtuous self-reinforcing circle of technology-component improvements and cost reductions.
- EVs hold great promise to replace ICEVs affordably for a number of on-road applications, eliminating petroleum dependence, improving local air quality and enabling GHG-emissions reductions, and improving driving experiences.
- Forecasting the future, including technology adoption, remains a daunting task. However, this detailed review paints a positive picture for the future of EVs across a number of on-road applications.
2. Status of electric-LDV market and future projections
This section provides a current snapshot of the electric-LDV market in a global and U.S. context, but focuses on the latter. The global rate of adoption of electric LDVs has increased rapidly since the mid-2010s 13 . By the end of 2019, the global EV fleet reached 7.3 million units—up by more than 40% from 2018—with more than 1.25 million electric LDVs in the U.S. market alone (IEA 2020 ). EV sales totaled more than 2.2 million in 2019, exceeding the record level that was attained in 2018, despite mixed performances in different markets. Electric-LDV sales increased in Europe and stagnated or declined in other major markets, particularly in China (with a significant slowdown due to changes in Chinese subsidy policy in July 2019), Japan, and U.S. U.S. EV adoption varies greatly geographically—nine counties in California currently see EVs accounting for more than 10% of sales (8% on average for California as a whole), but national-level sales remain at less than 3% (Bowermaster 2019 ). BEV sales exceeded those of plug-in hybrid electric vehicles (PHEVs) in all regions.
The rapid increase in EV adoption is underpinned by three key pillars:
- (a) Improvements and cost reductions in battery technologies, which were enabled initially by the large-scale application of lithium-ion batteries in consumer electronics and smaller vehicles (e.g. scooters, especially in China, IEA 2017 ). These developments offer clear and growing opportunities for EVs and HEVs to deliver a reduced total cost of ownership (TCO) in comparison with ICEVs.
- (b) A wide range of supportive policy instruments for clean transportation solutions in major global markets (Axsen et al 2020 ), which are mirrored by private-sector investment. These developments are driven by environmental goals (IPCC 2014 ), including reduction of local air pollution. These policy instruments support charging-infrastructure deployment (Bedir et al 2018 ) and provide monetary (e.g. rebates and vehicle-registration discounts) and non-monetary (e.g. access to high-occupancy-vehicle lanes and preferred parking) incentives to support EV adoption (IEA 2018a , AFDC 2020 ).
- (c) Regulations and standards that support high-efficiency transportation solutions and reduce petroleum consumption (e.g. fuel-economy standards, zero-emission-vehicle mandates, and low-carbon-fuel standards). These regulations are being supported by technology-push measures, consisting primarily of economic instruments (e.g. grants and research funds) that aim to stimulate technological progress (especially batteries), and market-pull measures (e.g. public-procurement programs) that aim to support the deployment of clean-mobility technologies and enable cost reductions due to technology learning, scale, and risk mitigation.
Transport electrification also has started a virtuous self-reinforcing circle. Battery-technology developments and cost reductions triggered by EV adoption provide significant economic-development opportunities for the companies and countries intercepting the battery and EV value chains. Adoption of alternative vehicles both is enabled and constrained by powerful positive feedback arising from scale and learning by doing, research and development, consumer experience and familiarity with technologies (e.g. neighborhood effect), and complementary resources, such as fueling infrastructure (Struben and Sterman 2008 ). In this context, more diversity in make and model market offerings is supporting vehicle adoption. As of April 2020, there are 50 EV models available commercially in U.S. markets (AFDC 2020 ), and ∼130 are anticipated by 2023 (Moore and Bullard 2020 ).
Measures that support transport electrification have been, and increasingly shall be, accompanied by policies that control for the unwanted consequences. Thus, the measures need to be framed in the broader energy and industry contexts.
When looking at the future, EV-adoption forecasts remain highly uncertain. Technology-adoption projections are used by a number of stakeholders to guide investments, inform policy design and requirements (Kavalec et al 2018 ), assess benefits of previous and ongoing efforts (Stephens et al 2016 ), and develop long-term multi-sectoral assessments (Popp et al 2010 , Kriegler et al 2014 ). However, projecting the future, including technology adoption, is a daunting task. Past projections often have turned out to be inaccurate. Still, progress has been made to address projection uncertainty (Morgan and Keith 2008 , Reed et al 2019 ) and contextualize scenarios to explore alternative futures in a useful way. Scenario analysis is used largely in the energy-environment community to explore the possible implications of different judgments and assumptions by considering a series of 'what if' experiments (BP 2019 ).
Adoption of advanced technologies historically has been underestimated in modeling and analysis results (e.g. Creutzig et al 2017 ), which fail to capture the rapid technological progress and its impact on sales. Historical experiences suggest that technology diffusion, while notoriously difficult to predict, can occur rapidly and with an extensive reach (Mai et al 2018 ). Projecting personally owned LDV sales is particularly challenging because decisions are made by billions of independent (not necessarily rational) decision-makers valuing different vehicle attributes based on incomplete information (e.g. misinformation and skepticism toward new technologies) and limited financial flexibility.
Many studies make projections for future EV sales (see figure 1 for a comparison of different projections). Some organizations (e.g. Energy Information Administration [EIA]) historically have been conservative in projecting EV success, mostly due to scenario constraints and assumptions. Still, U.S. EV-sales projections from EIA in recent years are much higher than in the past. Others (e.g. BloombergNEF and Electric Power Research Institute [EPRI]) consistently have been more optimistic in terms of EV sales and continue to adjust sales projections upward. Policy ambition for EV adoption is also optimistic. For example, in September 2020, California passed new legislation that requires that by 2035 all new car and passenger-truck sales be zero-emission vehicles (and that all medium- and heavy-duty vehicles be zero-emission by 2045) (California, 2020). Projected EV sales and outcomes from major energy companies vary widely, ranging from somewhat limited EV adoption (e.g. ExxonMobil) to full market success (e.g. Shell). A survey from Columbia University (Kah 2019 ) considers 17 studies and shows that 'EV share of the global passenger vehicle fleet is not projected to be substantial before 2030 given the long lead time in turning over the global automobile fleet' and that 'the range of EVs in the 2040 fleet is 10% to 70%'. The studies compared in figure 1 show an even greater variability for 2050 projections, ranging from 13% to 100% of U.S. EV adoption for LDVs.
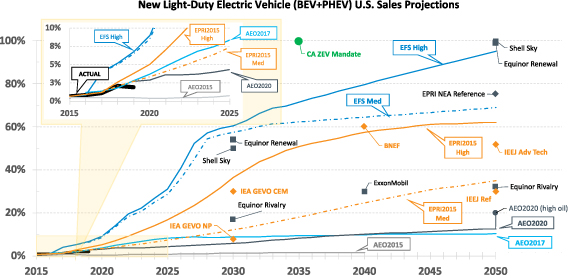
Figure 1. Electric LDV (BEV and PHEV) new sales projections from numerous international sources. Unless otherwise noted, data refer to new U.S. sales. AEO2015 = EIA Annual Energy Outlook 2015, Reference Scenario. AEO2017 = EIA Annual Energy Outlook 2017, Reference Scenario. AEO2020 = EIA Annual Energy Outlook 2020, Reference Scenario. AEO2020HO = EIA Annual Energy Outlook 2020, High Oil Scenario. EFS Med = National Renewable Energy Laboratory (NREL) Electrification Futures Study, Medium Scenario. EFS High = NREL Electrification Futures Study, High Scenario. EPRI Med = EPRI Plug-in Electric Vehicle Projections: Scenarios and Impacts, Medium Scenario. EPRI High = EPRI Plug-in Electric Vehicle Projections: Scenarios and Impacts, High Scenario. EPRI NEA = EPRI National Electrification Assessment, Reference Scenario. GEVO NP = IEA Global EV Outlook 2019, New Policies Scenario. GEVO CEM = IEA Global EV Outlook 2019, Clean Energy Ministerial 30@30 Campaign Scenario. BNEF = BloombergNEF EV Outlook 2020. Equinor Riv = Equinor 2019 Energy Perspectives, Rivalry Scenario. Equinor Ren = Equinor 2019 Energy Perspectives, Renewal Scenario. Shell Sky = Shell Sky Scenario. ExxonMobil = 2019 ExxonMobil Outlook for Energy. IEEJ Ref = The Institute of Energy Economics, Japan. 2019 Outlook, Reference Scenario (global sales). IEEJ Adv = The Institute of Energy Economics, Japan. 2019 Outlook, Advanced Technologies Scenario (global sales). CA ZEV Mandate = California zero-emission vehicle (ZEV) Executive Order N-79-20 (September 2020).
Download figure:
The future remains uncertain, but there is a clear trend in projections of light-duty EV sales toward more widespread adoption as the technology improves, consumers become more familiar with the technology, automakers expand their offerings, and policies continue to support the market.
A number of studies analyze the drivers of EV adoption (Vassileva and Campillo 2017 , Priessner et al 2018 ) and highlight several barriers for EVs to achieve widespread success, including consumer skepticism for new technologies (Egbue and Long 2012 ); uncertainty around environmental benefits (consumers wonder whether EVs actually are green; see section 7 for more clarity on the environmental benefits of EVs) and continued policy support; unclear battery aging/resale value; high costs (Haddadian et al 2015 , Rezvani et al 2015 , She et al 2017 ); lack of charging infrastructure (Melaina et al 2017 , Narassimhan and Johnson 2018 ); range anxiety (the fear of being unable to complete a trip) associated with shorter-range EVs; longer refueling times compared to conventional vehicles (Franke and Krems 2013 , Neubauer and Wood 2014 ; Melaina et al 2017 ); dismissive and deceptive car dealerships (De Rubens et al 2018 ); and other EV-supply considerations, such as limited model availability and limited supply chains.
A recent review of 239 articles published in top-tier journals focusing on EV adoption draws attention to 'relatively neglected topics such as dealership experience, charging infrastructure resilience, and marketing strategies as well as identifies much-studied topics such as charging infrastructure development, TCO, and purchase-based incentive policies' (Kumar and Alok 2019 ). Similar reviews published recently focus on different considerations, such as market heterogeneity (Lee et al 2019a ), incentives and policies (Hardman 2019 , Tal et al 2020 ), and TCO (Hamza et al 2020 ). Other than some limited discussions on business models and TCO, the literature is focused on one side of the story, namely demand. However, the availability (makes and models) of EVs is extremely limited compared to ICEVs (AFDC 2020 ). This is justified, in part, by new technologies requiring time to be introduced, and, in part, by the higher manufacturer revenues associated with selling and providing maintenance for ICEVs. Moreover, slow turnover in legacy industry (Morris 2020 ) and other supply constraints can be a major barrier to widespread EV uptake (Wolinetz and Axsen 2017 , De Rubens et al 2018 ). Kurani ( 2020 ) argues that in most cases, 'Results of large sample surveys and small sample workshops mutually reinforce the argument that continued growth of PEV markets faces a barrier in the form of the inattention to plug-in electric vehicles (PEVs) of the vast majority of car-owning and new-car-buying households even in a place widely regarded as a leader. Most car-owning households are not paying attention to PEVs or the idea of a transition to electric-drive.'
3. EVs beyond light-duty applications
While much of the recent focus on vehicle electrification is with LDVs and small two- or three-wheelers (primarily in China), major progress also is being made with the electrification of medium- and heavy-duty vehicles. This includes heavy-duty trucks of various types, urban transit buses, school buses, and medium-duty vocational vehicles. As of the end of 2019, there were about 700 000 medium- and heavy-duty commercial EVs in use around the world (EV Volumes 2020 , IEA 2020 ).
A major challenge facing both manufacturers and end-users of medium- and heavy-duty vehicles is the diverse set of operational requirements and duty cycles that the vehicles encounter in real-world operation. When designing powertrain configurations and on-board energy-storage needs for new technologies, it is of critical importance to represent vehicle behavior accurately for different operations, including possible changes triggered by electrification (Delgado-Neira 2012 ). Medium- and heavy-duty vehicles can require a large number of powertrain and battery configurations, control strategies, and charging solutions. These needs depend on vehicle type (covering the full U.S. gross vehicle weight ratings [GVWR] spectrum from class 3 to class 8, 10 001–80 000 lb [4536–36 287 kg]), commercial operational situations and activities, and diverse drive cycles and charging opportunities (e.g. depot-based operations vs. long-haul). An example of this potential variability and its effect on the required battery capacity across multiple vehicle vocations is shown in figure 2 (Smith et al 2019 ).
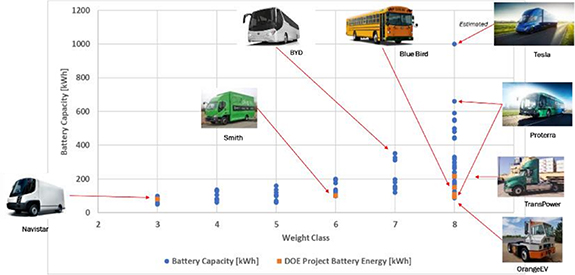
Figure 2. Battery capacity requirements vs. weight class for medium- and heavy-duty vehicles (Smith et al 2019 ).
Another example of the highly variable use cases for medium- and heavy-duty EVs shows energy efficiencies range between 0.8 kWh mile −1 and 3.2 kWh mile −1 (0.5–2.5 kWh km −1 ) (Gao et al 2018 ). If the on-board energy-storage needs for these vehicles are considered, assuming a daily operational range of between 50 miles and 200 miles (80–322 km), this results in battery-size requirements between 40 kWh and 640 kWh (assuming that the vehicle is recharged once daily). If additional charging strategies are considered (with their variability in expected charge times and associated power ratings), the range of vehicle-hardware and charging-infrastructure possibilities increases further. When adding variability across use cases with respect to temperature effects, battery-capacity degradation, payload, and road grade, it becomes clear that medium- and heavy-duty truck manufacturers face a significant challenge in designing, developing, and manufacturing systems that are able to meet the diverse operational requirements.
There are potential synergies between components of light-duty and medium- and heavy-duty electric vehicles. However, the requirements of medium- and heavy-duty vehicles place much greater burdens on powertrain components. The power and energy needs in heavy-duty applications are much larger than in light-duty applications. Heavy-duty vehicles could demand twice the peak power, four times the torque, and can consume more than five times the energy per mile (or km) driven compared to LDVs. In addition to using more energy per mile (or km) driven, typically, commercial vehicles drive many more miles (or km) per day, requiring much larger batteries and possibly much higher-power charging. Moreover, heavy-duty-vehicle users expect their vehicles to last more than a million miles, pointing to significantly higher durability requirements for heavy-duty-vehicle components (Smith et al 2019 ). Overall, these requirements, in combination with the needs for very high durability and very high-power drivelines and charging, may cause battery chemistries of heavy-duty vehicle batteries to diverge from those that are used in LDVs, hindering economies of scale. Demands for high efficiency, high power, and lower weight will put pressure on commercial vehicles to work at higher voltages than LDVs do. While LDVs are designed typically with powertrains that operate at a few hundred volts, it may be desirable to design large EVs with kilovolt powertrains. This will have a particularly significant impact on power electronics and could drive the development of wide-bandgap power electronics.
Historically, EVs have not been considered capable alternatives to heavy-duty diesel trucks (above 33 000 lb [14 969 kg] GVWR) due to high capital costs, high energy and power requirements, and weight and range-related battery constraints. International Council on Clean Transportation (ICCT), for example, suggests that while conventional EV-charging methods may be sufficient for small urban commercial vehicles, overhead catenary or in-road charging are required for heavier vehicles (Moultak et al 2017 ). Recent studies dispute this, anticipating a much greater opportunity for EVs to replace diesel trucks in the short-term, even for long-haul applications (Mai et al 2018 , McCall and Phadke 2019 , Borlaug et al Forthcoming ), but the potential for battery-electric models to work well in long-haul applications has yet to be established (NACFE 2018 ). Studies show that a significant amount of payload capacity will be consumed by batteries, potentially up to 7 tons or 28% of capacity in a truck with a 500 mile (805 km) range with 1100 kWh battery capacity (Burke and Fulton 2019 ). Thus, batteries would reduce significantly the amount of cargo that can be carried. Other studies suggest this could be much less―on the order of 4% of lost payload capacity for 500 mile range (805 km) trucks and with overall lower TCO than diesel trucks (Phadke et al 2019 ). For short-haul applications, such as port drayage and regional or local deliveries, EVs appear well suited and battery weight may not affect the cargo or payload capacity adversely. Several heavy-duty battery-electric trucks for short- and medium-haul applications have been developed and tested in recent years by Balqon, Daimler Trucks NA, Peterbilt, TransPower, Tesla, US Hybrid, Volvo, and others (AFDC 2020 ).
Urban buses are also a major emerging market for electrification. In California, Innovative Clean Transit rules require transit agencies to transition completely to zero-emission technologies (batteries or fuel cells), with all new bus purchases being zero-emission by 2029 (CARB 2018 ). Eight of the ten largest transit agencies in California already are adopting zero-emission technologies into their fleets (CARB 2018 ). In a comparative study of urban buses running on diesel, compressed natural gas, diesel hybrid, fuel cells, and batteries, the battery buses are estimated to have the lowest CO 2 emissions in both California and Finland bus duty cycles at the time of the study (Lajunen and Lipman 2016 ). This study also shows that battery buses have only slightly higher overall costs per mile (or km) than fossil-fuel-based alternatives. Future projections out to 2030 show that electric buses have the lowest overall life-cycle costs, especially when CO 2 costs are included (Lajunen and Lipman 2016 ).
Medium-duty delivery vehicles (typically 10 000–33 000 lb [4536–14 969 kg] GVWR) are another attractive emerging area for electrification. The goods-delivery market is growing at approximately 9% per year in recent years, with a projected $343 billion global industry value in 2020 (Accenture 2015 ). The 'last mile' delivery vehicles that are needed for this market are undergoing changes and present good opportunities for electrification. Amazon, for example, has announced plans to purchase 100 000 custom-designed Rivian electric delivery vans by 2030, with 10 000 of the vehicles delivered by late 2022 (Davies 2019 ).
A significant challenge with electrifying these heavy- and medium-duty vehicles revolves around the installation of the required charging infrastructure (either at depots or along highways). While LDVs typically charge at power levels of 3 kW–10 kW, and potentially 50 kW–250 kW with DC fast chargers (DCFCs), a heavy-duty vehicle may require higher-power charging, depending on its duty cycle. Fleets of these vehicles charging in one location, such as a truck depot or travel center, may require several megawatts of power. This requires expensive charging infrastructure, potentially including costly and time-consuming distribution-grid upgrades, to provide the higher voltage and current levels that are needed. For example, a single 350 kW DCFC that may be suitable for heavy-duty applications costs almost $150 000 today (Nelder and Rogers 2019 , Nicholas 2019 ). These costs would, in turn, impact the business case for vehicle electrification. Potential costs of grid upgrades to support these new electrical loads would be additional expenses that may or may not be supported by the local utility, depending on the circumstances. To enable reliable, low-cost charging, which is crucial when considering the TCO for a fleet owner, the installation and operational costs of the charging infrastructure must be optimized, requiring engagement with power-supply stakeholders.
4. Batteries, power electronics, and electric machines
Electrification is a key aspect of modern life, and electric motors and machines are prevalent in manufacturing, consumer electronics, robotics, and EVs (Zhu and Howe 2007 ). One reason for the recent success and rise in adoption of EVs is the use of advanced lithium-ion batteries with improved performance, life, and lower cost. Improved energy and power performance, increased cycle and calendar life, and lower costs are leading to EVs with longer electric range and better acceleration at lower cost premia that are attracting consumers. This section summarizes the state-of-the-art for batteries and for power electronics, electric machines, and electric traction drives in terms of cost, performance, power and energy density, and reliability, and highlights some research challenges, pathways, and targets for the future.
4.1. Batteries
Over the last 10 years, the price of a lithium-ion battery pack has dropped by almost 90% from over $1000 kWh −1 in 2010 to $156 kWh −1 at the end of 2019 (BloombergNEF 2020 ). Meanwhile, the specific energy of a lithium-ion battery cell has almost doubled from 140 Wh kg −1 to 240 Wh kg −1 during that same window of time (BloombergNEF 2020 ). The improvement in performance and cost comes mainly from engineering improvements, use of materials with higher capacities and voltages, and development of methods to increase stability for longer life and improved safety. Improvements in cell, module, and pack design also help to improve performance and lower costs. Increases in manufacturing volume due to EV sales contribute significantly to cost reductions (Nykvist and Nilsson 2015 , Nykvist et al 2019 ). However, further reductions in battery costs, along with a reduction in the cost of electric machines and power electronics, are needed for EVs to achieve purchase-price parity with ICEVs. This parity is estimated by U.S. Department of Energy (DOE) to be achieved at battery costs of ∼$100 kWh −1 (preferably less than $80 kWh −1 ) (VTO, 2020 ). At that point, EVs should have both a purchase- and a lifetime-operating-cost benefit over ICEVs. Such cost benefits are likely to trigger drastic increases in EV sales. Figure 3 shows the observed price of lithium-ion battery packs from 2010 to 2018, as well as estimated prices through 2030. BloombergNEF projects that by 2024 the price for original equipment manufacturers (OEMs) to acquire battery packs will go below $100 kWh −1 and reach ∼$60 kWh −1 by 2030 if high levels of investments continue in the future (BloombergNEF 2020 ).
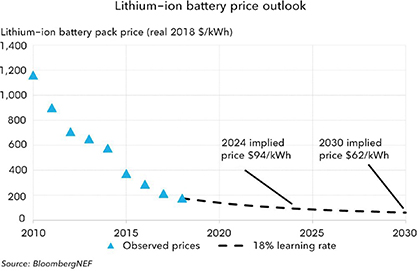
Figure 3. Evolution of battery prices over the last 10 years and future projections (Goldie-Scot 2019 ). BloombergNEF 2019.
The typical anode material that is used in most lithium-ion EV batteries is graphite (Ahmed et al 2017 ). Research is underway to utilize silicon, in addition to graphite, due to its higher specific-energy capacity. For cathodes, there is more variety (Lee et al 2019 , Manthiram 2020 ). Consumer electronics such as mobile phones and computers almost exclusively have used lithium cobalt oxide, LiCoO 2 , due to its high specific-energy density (Keyser et al 2017 ). Most EV manufacturers (except Tesla) have avoided using LiCoO 2 in EVs due to its high cost and safety concerns. Lithium iron phosphate also has been used for electric cars and buses because of its long life and better safety and power capabilities. However, due to its low specific-energy density (110 Wh kg −1 ) when paired with a graphite anode, lithium iron phosphate is not used commonly for light-duty EVs in U.S. In recent years, battery makers and vehicle OEMs have moved to lithium nickel manganese cobalt oxides (NMC) with varying ratios of the three transition metals. Initially, OEMs used NMC111 (the numbers represent the molar fractions of nickel, manganese, and cobalt, which are equal in this case), but they have transitioned to NMC532 and utilize NMC622 now while working to stabilize the NMC811 cathode structure. The goal is eventually to reduce the amount of cobalt in the cathode to less than 5% and perhaps even eliminate the use of cobalt. The use of these cathodes with higher specific-energy density and less cobalt leads to lower battery cost per unit energy ($ kWh −1 ). Table 1 shows the specific energy and estimated (bottom-up) cost from Argonne National Laboratory's BatPaC Battery Performance and Cost model (Ahmed et al 2016 ) based on large-volume material processing, cell manufacturing, and pack manufacturing.
Table 1. Calculated specific energy and cost of advanced lithium-ion batteries with different cathode/anode chemistries. Numbers are from BatPaC (Ahmed et al 2016 ) and are intended for relative comparison only. Final values can change depending on the components used and production volume, and costs reported do not reflect what a negotiated price could be between a battery and EV maker.
The cost of batteries is expected to decline in the future due to improved capacity of materials (such as Si anodes), increased percentage of active material components, use of lower-cost elements (no cobalt), improved packaging, and continued automation to increase yield while leading to a longer electric range. However, price increases for certain metals such as Ni and Li could prevent achieving those lower-battery-cost projections. Moreover, different battery chemistries can lead to very different costs and specific energies. For example, table 1 shows results obtained from bottom-up calculations with Argonne National Laboratory's BatPaC Battery Performance and Cost Model (Ahmed et al 2016 ), for a 100 kWh battery pack showing great variability in battery cost and performance for different chemistries.
Opportunities to improve performance and reduce costs further are being pursued in a number of major research areas. The battery community is investigating a number of materials, with the aim of reducing the cost and increasing the energy density of battery systems (Deign and Pyper 2018 ). Future work will involve utilizing silicon (Salah et al 2019 ) or lithium metal (Zhang et al 2020 ) as the anode while utilizing high-energy cathodes, such as NMC811 or lithium sulfur (Zhu et al 2019 ). Reducing the amount of critical material in lithium-ion batteries, especially cobalt, is an opportunity to lower the cost of batteries and improve supply-chain resilience. The private and public sectors are working toward developing new cathode materials along these lines (Li et al 2009 , 2017b ). Research and development (R&D) projects are underway to develop infrastructure and recycling technologies to collect batteries and recover the key battery materials economically and environmentally (Harper et al 2019 ). Reuse of end-of-life batteries from EVs would delay the need for additional battery materials, which should have positive environmental benefits (Neubauer et al 2012 ). Different battery technologies also are being explored. To increase energy density, reduce cost, and improve safety, the battery community is pursuing development of solid-state batteries with solid-state electrolytes (Randau et al 2020 ) that have ionic conductivities approaching those of today's liquid electrolyte systems. Solid-state lithium batteries enable the use of metallic lithium anodes, together with solid electrolytes and high-energy cathodes (such as high-nickel NMC or sulfur). Lithium-metal batteries based on solid electrolytes can, in principle, alleviate the safety concerns with current lithium-ion batteries with a flammable organic electrolyte. The main challenges facing lithium-metal anodes are dendritic growth, especially at low temperatures and higher current rates. Dendritic growth could lead to short circuit and thermal runaway and low Coulombic efficiency leading to poor cycle life (Xia et al 2019 ). Slow ion transport through the solid-state electrolyte leading to low power densities and manufacturing challenges, including poor mechanical integrity, pose additional challenges. Significant R&D activities are focused on developing solid-state electrolytes that prevent dendrite growth, have high ionic conductivity, good voltage-stability windows, and low impedance at the electrode–electrolyte interface. Recent cathode formulations in Li-S cells overcome the polysulfide problem, which could lead to lower efficiency and cycle life. Nevertheless, the deployment of cells with lower electrolyte-to-sulfur ratios for scale-up to large sizes is a remaining challenge. It may take another 5 to 10 years to mass-produce solid-state lithium batteries for EV applications.
As is discussed in section 5 , a network of fast chargers and batteries that can handle high charging-power rates is needed to address any potential barriers to widespread EV adoption. Research is focusing on developing batteries that can be charged very quickly (e.g. 80% of capacity in less than 15 min). A number of challenges to high-power charging, such as lithium plating, thermal management, and poor cycle life, need to be addressed (Ahmed et al 2017 ; DOE 2017 , Michelbacher et al 2017 ). Significant efforts also have focused on developing electrochemical and thermal modeling of batteries for EV applications (Kim et al 2011 , Chen et al 2016 , Keyser et al 2017 , Zhang et al 2017 ) to improve battery lifetime and efficiency in real-world applications. These efforts include lifetime-estimation and degradation modeling under different real-world climate and driving conditions (Hoke et al 2014 , Neubauer and Wood 2014 , Liu et al 2017b , Harlow et al 2019 , Li et al 2019 ); simplified models for control and diagnostics (e.g. state-of-charge estimation) (Muratori et al 2010 , Fan et al 2013 , Cordoba-Arenas et al 2015 , Bartlett et al 2016 ); and developing effective thermal management and control strategies (Pesaran 2001 , Serrao et al 2011 ).
Besides EV applications, batteries can offer energy-storage solutions for hybrid- or distributed-energy systems. These solutions include the use of batteries in integrated configurations with wind or solar photovoltaic (PV) systems or with EV fast-charging stations (Bernal-Agustín and Dufo-Lopez 2009 , Badwawi et al 2015 , Muratori et al 2019a ). Batteries also can provide stabilization and flexibility and can improve resilience and efficiency for power systems in general, especially for critical services or when a high share of variable power generation (e.g. from solar or wind) is expected (Divya and Østergaard 2009 , Denholm et al 2013 ; De Sisternes et al 2016 ). Lithium-ion batteries that have been developed for EV applications have found their way into stationary applications (Pellow et al 2020 ) because of their lower cost and modularity compared to other energy-storage technologies (Chen et al 2020 ). Moreover, EV batteries can be reused or repurposed at the end of their 'vehicle life' (usually considered when energy storage capacity drops below 70%–80% of the original nominal value, (Podias et al 2018 )) for stationary applications, improving their economic and environmental performance (Assuncao et al 2016 , Ahmadi et al 2017 , Martinez-Laserna et al 2018 , Olsson et al 2018 , Kamath et al 2020 ).
4.2. Power electronics, electric machines, and electric-traction-drive systems
While batteries are playing a key role in the rise of EVs, power electronics and electric motors and machines are also key components of an EV powertrain. Traditionally, the motor and power electronics drive were separate components in an EV. However, recent trends toward integration promise to deliver benefits in terms of increased power density, lower losses, and lower costs compared with separate motor and motor-drive solutions (Reimers et al 2019 ). Figure 4 shows the 2020 power density for power electronics, electric machines, and electric-traction-drive system from some example commercial vehicles as well as the 2025 DOE and U.S. DRIVE Partnership targets for near-term improvements (U.S. DRIVE 2017 , Chowdhury et al 2019 ). Commercially available vehicles exceed the 2020 power-density target. However, the 2025 target is at least a factor of six to eight higher than current commercial baselines. U.S. DRIVE Partnership also proposes electric-traction-drive-cost targets for 2020 and 2025: $8 kW −1 and $6 kW −1 , respectively, both of which are challenging targets (U.S. DRIVE 2017 , Chowdhury et al 2019 ). The authors are not aware of commercial systems meeting the 2020 target, and the 2025 target represents a further 33% reduction.
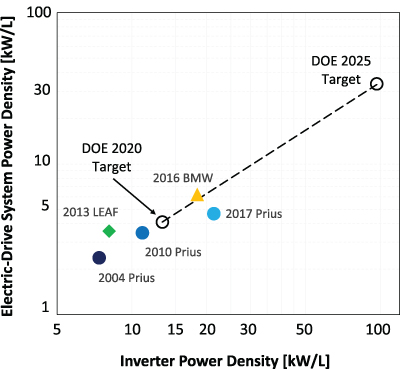
Figure 4. Integrated electric-drive system and inverter power density for several commercial light-duty vehicles and DOE targets (data from U.S. DRIVE 2017 , Chowdhury et al 2019 ).
Improvements in compact power electronics and electric machines are applicable to novel emerging wheel-integrated solutions as well (Iizuka and Akatsu 2017 , Fukuda and Akatsu 2019 ). The development of advanced electric traction drive with improved efficiency is a strategy for increasing the range of electric-drive vehicles. In addition to this, chassis light-weighting is another strategy that is being pursued by the industry and the research community for increasing EV driving ranges. There are several technical challenges in meeting the DOE power-density targets (shown in figure 4 ). Challenges in meeting related DOE cost targets remain as well. A range of integration approaches are proposed in the literature, including surface mounting the power electronics on the motor housing (Nakada, Ishikawa, and Oki 2014 ), mounting on the motor stator iron (Wheeler et al 2005 ), and piecewise integration. Piecewise integration involves modularizing both power modules and machine stators into smaller units (Brown et al 2007 ). In all cases, the close physical positioning of the power electronics relative to the machine and the associated harsh thermal environment necessitate new concepts related to the active cooling of both components. A first strategy may be to isolate the power electronics from the machine thermally using parallel cooling mechanisms (Wheeler et al 2005 ). Another approach may be to use a fully integrated, series-connected, active-cooling loop (Tenconi et al 2008 , Gurpinar et al 2018 ). In either case, cost benefits may be realized through the possible elimination or combination of cooling loops. Significant research also has been focused on reducing rare-earth and heavy-rare-earth materials within the electric machines because that is an additional important pathway to reduce costs (U.S. DRIVE 2017 ).
Higher levels of integration go hand-in-hand with the utilization of wide-bandgap (WBG) semiconductor devices, which may be used at higher operational temperatures (e.g. >200 °C versus 150 °C for silicon) with reduced switching loss (Millán et al 2014 ). However, the adoption of WBG devices requires new packaging technologies to support the end goals of high temperature, high frequency, higher voltages, and more compact footprints. High-performance electrical interconnects (Cheng et al 2013 ), die-attach (Liu et al 2020 ), encapsulation (Cao et al 2010 ), and power-module-substrate technologies (Stockmeier et al 2011 ), along with thermal management and reliability of these technologies (Moreno et al 2014 , Paret et al 2016 , 2019 ), are critical aspects to consider. The new materials, devices, and components must be cost-effective and high-temperature-capable to be compatible with WBG devices. The downsizing of passive electrical components is another added benefit of adopting WBG devices and a further necessity for integrated machine-drive packaging solutions. Fortunately, the higher switching frequencies that are supported by WBG devices enable the downsizing of both the inductors and capacitors found in a traditional power-control unit (Hamada et al 2015 ). The development of economically viable and high-temperature-capable passives, capacitors in particular (Caliari et al 2013 ), is an area of great interest.
Besides EV applications, power electronics and electric machines with low cost, high performance, and high reliability are important for numerous energy-efficiency and renewable-energy applications, such as solar inverters, generators and electric drives for wind, grid-tied medium-voltage power electronics, and sensors and electronics for high-temperature geothermal applications (PowerAmerica 2020 ).
5. Charging infrastructure
Infrastructure planning and deploying an ecosystem of cost-effective and convenient public and private chargers is central to supporting EV adoption (CEM 2020 ). The lack of a sufficient refueling infrastructure has hampered many past efforts to promote alternatives to petroleum fuels (McNutt and Rodgers 2004 ). Extensive research is being done to address the diverse challenges that are posed by a transition from fossil-fuelled ICEVs to EVs and the special role of charging infrastructure in this transition (Muratori et al 2020b ).
At the end of 2019, there were an estimated 7.3 million EV chargers (or plugs) worldwide, of which almost 0.9 million were public, including approximately 264 000 public DCFCs (81% in China) (IEA 2020 ). Significant government support and private investments are helping to expand the network of public charging stations worldwide. With about 7.2 million light-duty BEVs on the road, there is about one public charger per 10 light-duty BEVs, and most vehicles have access to a residential charger. However, the number of public chargers per BEV varies widely among the 10 countries with the most BEVs (figure 5 ) because of different strategies for deploying fast versus slow public chargers. In addition to these LDV chargers, IEA estimated there are 184 000 fast chargers dedicated to electric buses (95% in China).
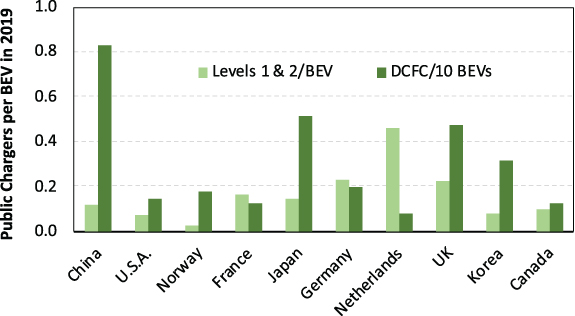
Figure 5. Public charging availability by country in 2019, measured as Level-1 and Level-2 chargers per BEV and DCFC per 10 BEVs (Data from IEA 2020 ).
Studies show consistently that today's EVs do the majority (50%–80%) of their charging at home, followed by at work (15%–25% when workers use their vehicles to commute), and using public chargers (only about 5% of charging) (Hardman et al 2018 ). PHEVs conduct more charging at home than BEVs do, and they rely more on level-1 charging (Tal et al 2019 ). While single-household detached residences readily can accommodate level-1 or -2 charging, multi-unit dwellings require curbside public charging or installations in shared parking facilities (Hall and Lutsey 2017 ). Historical data on the charging behavior of California BEV owners reveals that 11% of their charging sessions were at level 1, 72% were at level 2, and 17% used DCFCs (Tal et al 2019 ). Use of DCFCs is lowest for BEVs with less than 100 miles (161 km) of range, highest for medium-range BEVs, and lower again for BEVs with ranges of 300 miles (483 km) or more.
5.1. Charging-siting modeling
Public charging infrastructure is clearly important to EV purchasers and supports EV sales by adding value (Narassimhan and Johnson 2018 , Greene et al 2020 ). However, how best to deploy charging infrastructure, in terms of numbers, types, locations, and timing remains an active area for research (Ko et al 2017 , Funke et al 2019 provide reviews). The literature includes many examples of geographically and temporally detailed models to optimize the location, number, and types of charging stations (e.g. Wood et al 2017 , Wu and Sioshansi 2017 , Zhao et al 2019 ). Geographically and temporally detailed data recording the movements of PEVs and their charging behavior are scarce. With few exceptions (e.g. Gnann et al 2018 ), simulation analyses rely on conventional ICEV databases (e.g. Dong et al 2014 , Wood et al 2015 , 2018 ), which do not reflect the changes PEV owners will make to maximize the utility of PEVs.
Given the importance of home charging, access to chargers for on-street parking in residential areas comprising attached or multi-unit dwellings is likely to be essential for PEVs to be adopted at large scale. Grote et al ( 2019 ) employ heuristic methods with geographical-information systems to locate curbside chargers in urban areas using a combination of census and parking data. The works of Nie and Ghamami ( 2013 ), Ghamami et al ( 2016 ), and Wang et al ( 2019 ) are examples of the variety of optimization methods that are applied to design DCFC networks to support intercity travel. Despite these examples, applied research is hindered by the scarcity of data on long-distance vehicle travel by PEVs (Eisenmann and Plötz 2019 ). Jochem et al ( 2019 ) estimate that 314 DCFC stations could provide minimum coverage of EU intercity routes with approximately 0.7 charging points per 1000 BEVs. Using a database of simulated U.S. intercity travel, He et al ( 2019 ) employ a mixed-integer model to optimize the location and number of DCFCs. They conclude that 250 stations could serve 98% of the long-distance miles of BEVs with ranges of 150 miles (241 km) or greater but only 73% of the long-distance miles of 100 mile range (161 km range) BEVs. Similarly, Wood et al ( 2017 ) estimate that 400 DCFC stations are required to cover the U.S. interstate-highway network with a 40 mile (64 km) spacing between stations. Others consider the optimal location of dynamic, wireless charging in combination with stationary charging (Liu and Wang 2017 ).
Optimization models for locating chargers to support commercial PEV fleets also appear in the literature (Jung et al 2014 , Shahraki et al 2015 ). In the future, if vehicle sharing becomes much more common, the downtime for charging could be an important disadvantage for PEVs. Using an integer model to optimize station allocation and PEV assignment, Roni et al ( 2019 ) find that charging time represents 72%–75% of vehicle downtime but that charging time could be reduced by almost 50% by optimal deployment of charging stations.
5.2. Beyond LDV charging
The electrification of medium- and heavy-duty commercial trucks and buses introduces unique charging and infrastructure requirements compared to those of LDVs. These requirements stem from the significantly higher battery capacities required on-board the vehicles, potentially shorter charging-dwell times (due to the in-service time requirements of the vehicles), and the potential of large facility charging loads (due to multiple trucks or buses charging in one location). One challenge is to understand the costs associated with the multitude of charging scenarios for commercial vehicles for current operations as well as future operations. It is expected that on-road freight vehicle miles (or km) traveled will increase by 75% from 2012 to 2045 (McCall and Phadke 2019 ). This increase may bring about new business models and potentially new charging-infrastructure approaches to meet this demand with electrified trucks. California's Innovative Clean Transit regulation, which will require California transit agencies to adopt zero-emission buses by 2040, is likely to drive large charging-infrastructure investments for buses (CARB 2018 ).
Today's commercial diesel-powered trucks in small fleets typically are fueled at publicly available on-road fueling stations, while nearly half of trucks in fleets of 10+ vehicles use company-owned facilities (Davis and Boundy 2020 ). Likewise, commercial EVs are charged primarily in fleet-owned facilities as their daily schedule allows (most often overnight). This depot-charging approach, which enables seamless integration of EVs into fleet logistics, might limit the electrification of some vehicle segments in the long term due to the battery capacity that is needed to satisfy their daily-range requirements (the need to complete their full-day function) and return to the facility to recharge fully 14 . Some studies suggest that long-haul battery-electric trucks are technically feasible and economically compelling (Phadke et al 2019 ) while others are more skeptical (Held et al 2018 ). Publicly available, high-power charging or en-route charging infrastructure for commercial vehicles could enable electrification for longer-distance vehicles (by enabling smaller on-board battery-capacity needs), but this scenario has cost challenges. En-route, high-power charging of over 1 MW might be needed to enable 500 miles (805 km) or more of daily driving. Installation of a 20 MW truck-charging station in California (capable of multiple 1.5 MW charge events for heavy-duty freight vehicles) is estimated to cost as much as 15 million USD. McCall and Phadke ( 2019 ) estimate that as many as 750 of these stations are needed to electrify the fleet of California Class-8 combination trucks. Charging commercial vehicles at depots requires additional infrastructure costs to install lower-power EV-supply equipment networks (e.g. 50 kW–100 kW) capable of charging multiple vehicles at these lower rates. These depot charging systems also will challenge existing facility electrical systems by adding a significant load that was not planned previously at the facility (Borlaug et al Forthcoming ).
5.3. Economics of public charging
PEV-charging economics vary with location and station configuration and depend critically on equipment and installation costs and retail electricity prices, which are dependent on utilization (Muratori et al 2019b , Borlaug et al 2020 ). In the early stages of market development, when there are relatively few vehicles, future demand is uncertain, and most charging is done at an EV's home base (Nigro and Frades 2015 , Madina et al 2016 ). Public charging stations tend to be lightly used during these initial stages (e.g. INL 2015 ), which poses a difficult challenge for private investment. Understanding and quantifying the value of public charging is hindered by lack of experience with PEVs on the part of consumers (Ito et al 2013 , Greene et al 2020 , Miele et al 2020 ) and the complexity of network effects in the evolution of alternative-fuel-vehicle markets (Li et al 2017a ). Nevertheless, it is likely that DCFCs will be profitable with sufficient demand. Considering vehicle ranges of between 100 km and 300 km and charging-power levels of between 50 kW and 150 kW, Gnann et al ( 2018 ) conclude that charger-usage fees could be between 0.05 € kWh −1 and 0.15 € kWh −1 in addition to the cost of electricity. The estimates were based on simulations with average daily occupancy of charging points of 10%–25% and peak-hour utilization of 20%–70%. In their simulations, utilization rates increase with increasing charger power and decrease with increased EV range. For intercity travel along European Union highways, Jochem et al ( 2019 ) estimate that a surcharge of 0.05 € kWh −1 of DCFC would make a minimal coverage of 314 stations (with 20 charge plugs each) profitable, even for station capital costs of one million EUR. He et al ( 2019 ) optimize DCFC locations along U.S. intercity routes and conclude that providing an adequate nationwide charging network for long-distance travel by 100 mile (161 km) range BEVs is more economical than increasing vehicle range and reducing the number of charging stations. Muratori et al ( 2019a ) consider a set of charging scenarios from real-world data and thousands of U.S. electricity retail rates. They conclude that batteries can be highly effective at mitigating electricity costs associated with demand charges and low station utilization, thereby reducing overall DCFC costs.
Early estimates show that the cost of public DCFC in U.S. can vary widely based on the station characteristics and level of use (Muratori et al 2019a ). Numerous new technology options are being explored to provide lower-cost electricity for light-duty passenger and medium- and heavy-duty commercial BEVs. Increasing the range of EVs through higher-power public charging stations as well as accommodating new potential BEV business models, such as transportation-network companies or automated vehicles, are driving new charging-technology solutions. Managed charging solutions that are available today can provide increased value to the BEV owner (lower electricity costs), charging station owner (lower operating costs), or grid operator (lower infrastructure-investment costs). For example, a managed-charging solution has been adopted and is currently in operation at a Santa Clara Valley Transportation Authority depot to charge a fleet of Proterra electric buses optimally to ensure minimal stress on the grid (Ross 2018 ).
5.4. Emerging charging technologies
Wireless charging, specifically high-power wireless charging (beyond level-2 power levels), could play a key role in providing an automated charging solution for tomorrow's automated vehicles (Lukic and Pantic 2013 , Qiu et al 2013 , Miller et al 2015 , Feng et al 2020 ). Wireless charging also can enable significant electric range for BEVs by providing en-route opportunity charging (static or dynamic charging opportunities). If a network of wireless charging options is available to provide convenient and fast en-route charging, it could help reduce the amount of battery that is needed on-board a vehicle and reduce the cost of ownership for a BEV owner. Wireless charging is being developed for power levels of up to 300 kW for LDVs, 500 kW for medium-duty vehicles, and 1000 kW for heavy-duty vehicles. Bidirectional functionality, improved efficiency, interoperability of different systems, improved cybersecurity, and increased human-safety factors continue to be developed (Ozpineci et al 2019 ).
Connectivity and communication advances will enable new BEV-charging infrastructure and managed charging solutions. However, emerging cybersecurity threats also are being identified and should be addressed. There are concerns associated with data exchange, communications network, infrastructure, and firmware/software elements of the EV infrastructure (Chaudhry and Bohn 2012 ), and new charging-system security requirements and protocols are being developed to address these concerns (ElaadNL 2017 ). New emulation and simulation platforms also are being developed to address these threats and help understand the consequences and value of mitigating cyberattacks that could affect BEVs, electric-vehicle-supply equipment, or the electric grid (Sanghvi et al 2020 ).
6. Vehicle-grid integration (VGI)
Connecting millions of EVs to the power system, as may occur in the coming decades in major cities, regions, and countries around the world, introduces two fundamental themes: (a) challenges to meet reliably overall energy and power requirements, considering temporal load variations, and (b) VGI opportunities that leverage flexible vehicle charging ('smart charging') or V2G services to provide power-system services from connected vehicles. Multiple studies, which are reviewed in detail below, investigate the potential load growth, impact on load shapes, and infrastructure implications of increased EV adoption. These works focus especially on impact on distribution systems and opportunities for flexible charging to reshape aggregate power loads. Mai et al ( 2018 ), for example, shows that in a high-electrification scenario, transportation might grow from the current 0.2% to 23% of total U.S. electricity demand by 2050. This growth would impact system peak load and related capacity costs significantly if not controlled properly. In-depth analytics indicate a complex decision framework that requires critical understanding of potential future mobility demands and business models (e.g. ride-hailing, vehicle sharing, and mobility as a service), technology evolution, electricity-market and retail-tariff design, infrastructure planning (including charging), and policy and regulatory design (Codani et al 2016 , Eid et al 2016 , Knezovic et al 2017 , Borne et al 2018 , Hoarau and Perez 2019 , Gomes et al 2020 , Muratori and Mai 2020 , Thompson and Perez 2020 ).
While accommodating EV charging at the bulk-power (generation and transmission) level will be different in each region, no major technical challenges or risks have been identified to support a growing EV fleet, especially in the near term (FleetCarma 2019 , U.S. DRIVE 2019 , Doluweera et al 2020 ). At the same time, many studies show that smart charging and V2G create opportunities to reduce system costs and facilitate VRE integration (Sioshansi and Denholm 2010 , Weiller and Sioshansi 2014 , IRENA 2019 , Zhang et al 2019 ). Therefore, charging infrastructure that enables smart charging (e.g. widespread residential and workplace charging) and alignment with VRE generation and business models and programs to compensate EV owners for providing charging flexibility are critical elements for successful integration of EVs with bulk power systems.
6.1. Impact of EV loads on distribution systems
At the local level, EV charging can increase and change electricity loads significantly, having possible negative impacts on distribution networks (e.g. cables and distribution transformers) and power quality or reliability (Khalid et al 2019 ). Residential EV charging represents a significant increase in household electricity consumption that can require upgrades of the household electrical system which, unless managed properly, may exceed the maximum power that can be supported by distribution systems, especially for legacy infrastructure and during times of high electricity utilization (e.g. peak hours and extreme days) (IEA 2018b ). The impact of EVs on distribution systems also is influenced by the simultaneous adoption of other distributed energy resources, e.g. rooftop PV panels. While this interdependency complicates assessing the impact of EV charging, Fachrizal et al ( 2020 ) show that the two technologies support one other. Similarly, Vopava et al ( 2020 ) show that line overloads caused by rooftop PV panels can be reduced (but not avoided) by increasing EV adoption and vice versa.
The impact of EV charging on distribution systems is particularly critical for high-power charging and in cases in which many EVs are concentrated in specific locations, such as clusters of residential LDV charging and possibly fleet depots for commercial vehicles (Saarenpää et al 2013 , Liu et al 2017a , Muratori 2018 ). Smart charging, by which EV charging is timed based on signals from the grid and electricity prices that vary over time, or other forms of control, can help to minimize the impact of EV charging on distribution networks. However, smart charging requires both appropriate business models and signals (with related communication and distributed-control challenges). The market for distribution-system operators to provide such services is not mature yet (Everoze 2018 , Crozier, Morstyn, and McCulloch 2020 ). Time-varying pricing schemes, which are effective at influencing the timing of EV charging (PG&E 2017 ), typically do not include any distribution-level considerations. Thus, while consumers are responsive to such signals, the business models to include distribution-level metrics still are lacking. Moreover, price signals are offered usually to a large consumer base with the intent of reshaping the overall system load. At the local level, however, multiple consumers responding to the same signal might cause 'rebound peaks' (Li et al 2012 , Muratori and Rizzoni 2016 ) that can overstress distribution systems, calling for coordination among consumers connected to the same distribution network (e.g. direct EV-charging control from an intermediate aggregator).
Charging of larger commercial vehicles and highway fast-charging stations typically involves higher power levels: DCFC is typically at 50 kW/plug today, but power levels are increasing rapidly. Commercial charging locations with multiple plugs co-located at a specific location may lead to possible MW-level loads, which is roughly equivalent to the peak load of a large hotel. Commercial DCFC may require costly upgrades to distribution systems that can impact the cost-effectiveness of public fast charging heavily, especially if stations experience low utilization (Garrett and Nelder 2016 , Muratori et al 2019b ). While charging timing and speed at commercial stations is less flexible (consumers want to charge and leave or commercial fleets must meet business requirements), business models are often already in place to incentivize curbing maximum peak power from commercial installations. For example, demand charges (a fixed monthly payment that is proportional to the peak power that is drawn during a given month) are fairly common in U.S. retail tariffs and provide a reason to limit peak power. Furthermore, Muratori et al ( 2019a ) show that distributed batteries can be effective at mitigating the cost associated with demand charges by up to 50%, especially for 'peaky' or low-utilization EV-charging loads. Batteries also can facilitate coupling EV-charging stations with local solar electricity production or can provide grid services (Megel, Mathieu, and Andersson 2015 ), generating additional revenue.
6.2. Value of managed ('smart') EV charging for power systems
The integration of EVs into power systems presents several opportunities for synergistic improvement of the efficiency and economics of electromobility and electric power systems. These synergies stem from two inherent characteristics of EVs and power systems. Demand response and other forms of demand-side flexibility can be of value for power-system planning and operations (Albadi and El-Saadany 2007 , 2008 , Su and Kirschen 2009 , Muratori et al 2014 ). Contemporaneously, most personal-vehicle driving patterns entail vehicle-use for mobility purposes a relatively small proportion of the time (Kempton and Letendre 1997 ). If EVs are grid-connected for extended periods of time, they can provide demand-side flexibility in the form of smart charging or V2G services. Such use of an EV can improve its economics by leveraging cheaper electricity at little incremental cost (e.g. the costs of monitoring, communication, and control equipment that are needed to manage smart charging). EVs can support grid planning and operations in a number of ways. Figure 6 summarizes the key support services that EVs can provide. These services include reducing peak load and generation-, transmission-, and distribution-capacity requirements, deferring system upgrades, providing load response, supporting power-system dispatch (including VRE integration and real-time energy and operating reserves), providing energy arbitrage, and supporting power quality and end retail consumers.
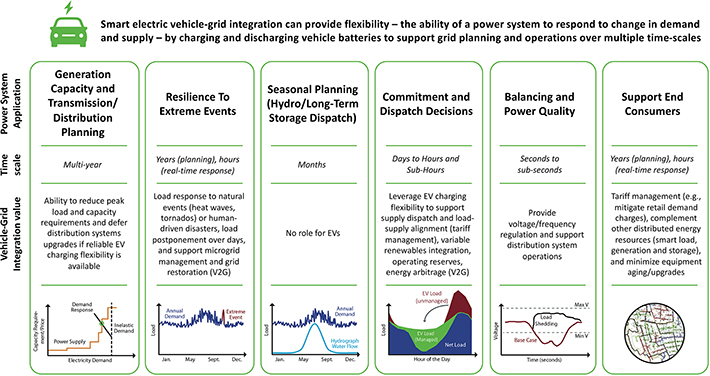
Figure 6. Summary of opportunities for EVs to provide demand-side flexibility to support power system planning and operations across multiple timescales.
Habib et al ( 2015 ) and Thompson and Perez ( 2020 ) provide detailed surveys of different potential uses of EVs for smart charging and V2G services. This includes active- and reactive-power services, load balancing, power-quality-related services (e.g. managing flicker and harmonics), retail-bill management, resource adequacy, and network deferral. In addition, Habib et al ( 2015 ) discuss different standards and technology needs relating to V2G services.
Kempton and Letendre ( 1997 ) provide the first description of the concept of EVs providing grid services, either in the form of smart charging or bidirectional V2G services (which can involve discharging EV batteries). Denholm and Short ( 2006 ) study the benefits of controlled overnight charging of PHEVs for valley-filling purposes. They demonstrate that with proper control of vehicle charging, up to 50% of the vehicle fleet could be electrified without needing new generation capacity to be built and at substantial savings compared to using liquid fuels for transportation. They show also that under conservative utility-planning practices, PHEVs could replace a significant portion of low-capacity-factor generating capacity by providing peaking V2G services. Tomić and Kempton ( 2007 ) examine the economics of using EVs for the provision of frequency reserves and demonstrate that such services can yield substantive revenues to vehicle owners in a variety of wholesale markets. Thompson and Perez ( 2020 ) conduct a meta-analysis of V2G services and value streams and find that power-focused services are of greater value than energy-focused services. They distinguish the two types of services based on the extent to which EV batteries must be discharged and degraded. Sioshansi and Denholm ( 2010 ) come to a similar conclusion in comparing the value of using PHEV batteries for energy arbitrage and operating reserves.
Another important synergy between EVs and power systems is using the flexibility of EV charging to manage the integration of VRE into power systems (Mwasilu et al 2014 , Weiller and Sioshansi 2014 ). Hoarau and Perez ( 2018 ) develop a framework for examining the synergies between EV charging and the integration of photovoltaic-solar resources into power systems. They find that the spatial footprint across which solar resources and EVs are deployed and the regulatory, policy, and market barriers to cooperation between solar resources and EVs are critical to realizing these synergies. Szinai et al ( 2020 ) find that controlled EV charging in California under its 2025 renewable-portfolio standards can reduce operational costs and renewable curtailment compared to unmanaged charging. They find that properly designed time-of-use retail tariffs can achieve some, but not all, of the benefits of controlled EV charging. They show also that these two approaches to managing EV charging (controlling EV charging directly and time-of-use tariffs) reduce the cost of infrastructure that is necessary to accommodate EV charging relative to a case of uncontrolled EV charging. Chandrashekar et al ( 2017 ) conduct an analysis of the Texas power system and find similar benefits of controlled EV charging in reducing wind-integration costs. Coignard et al ( 2018 ) show that under California's 2020 renewable-portfolio standards, controlled EV charging can deliver the same renewable-integration benefits that California's energy-storage mandate does but at substantially lower costs. They show that bidirectional V2G services deliver up to triple the value of controlled EV charging. Kempton and Tomić ( 2005 ) show that high penetrations of wind energy in U.S. could be accommodated at relatively low costs if 3% of the vehicle fleet provides frequency reserves and 8%–38% of the fleet provides operating reserves and energy-storage services to avoid wind curtailment. Loisel et al ( 2014 ) and Zhang et al ( 2019 ) conduct more forward-looking analyses of the synergies between EVs and renewables. The former examines German systems, and the latter examines California systems under potential renewable-deployment scenarios in the year 2030.
An important assumption underlying these works is that EV owners (or aggregations of EVs) are exposed to prices that signal the value of these services and that there are regulatory and business models that allow such services to be exploited (i.e. consumer are willing to engage in these programs and are compensated properly for providing flexible charging). Several pilot studies suggest that EV owners have interest in participating in utility-run controlled-charging programs and that a set of different compensation strategies beyond time-varying electricity pricing might maximize engagement (Geske and Schumann 2018 , Hanvey 2019 , Küfeoğlu et al 2019 , Delmonte et al 2020 ).
Niesten and Alkemade ( 2016 ) survey the literature on these topics and numerous European and U.S. pilot programs in terms of value generation for V2G services. They find that the ability of an aggregator to scale is related to its ability to develop a financially viable business model for V2G services. Another important consideration is the availability of control and communication technologies to manage EV charging based on power-system conditions. Key considerations in the design of control strategies are robustness in the face of uncertainty (e.g. renewable availability, EV-arrival times and charge levels upon arrivals, and EV-departure times), data privacy, and robustness to communication or other failures. Le Floch et al ( 2015a ), Le Floch et al ( 2015b ), ( 2016 ), develop a variety of distributed and partial-differential-equation-based algorithms for controlling EV charging. Rotering and Ilic ( 2011 ) develop a dynamic-optimization-based approach to control EV charging and bidirectional V2G services (with a focus on the provision of ancillary services). Donadee and Ilic ( 2014 ) develop a Markov decision process to optimize the offering behavior of EVs that participate in wholesale electricity markets to provide frequency reserves.
6.3. Remaining challenges for effective vehicle-grid integration at scale
There are still many challenges to tackle before smart charging and V2G can be deployed effectively at large scale. These challenges are linked to the technical aspects of VGI technology but also to societal, economic, security, resilience, and regulatory questions (Noel et al 2019a ). With regard to the technical challenges of VGI, existing barriers notably include battery degradation, charger availability and efficiency, communication standards, cybersecurity, and aggregation issues (Eiza and Ni 2017 , Sovacool et al 2017 , Noel et al 2019a ).
While the technical aspects of VGI are studied widely, this is much less the case for its key societal aspects. Societal issues include the environmental performance of VGI, its impact on natural resources, consumer acceptance and awareness, financing and business models, and social justice and equity (Sovacool et al 2018 ). There are also various regulatory and political challenges linked to clarifying the regulatory frameworks applicable to VGI as well as market-design issues, such as the proper valuation of VGI services and double taxation (Noel et al 2019a ) and the trade-offs between bulk power and distribution-system needs. Regulatory changes may be required to enable distribution-network operators and EV owners (or aggregators) to take a more active role in electricity markets. The Parker project, an experimental project on balancing services from an EV fleet, underlines some of the barriers to providing ancillary services, such as metering requirements (Andersen et al 2019 ). It is argued that insufficient regulatory action might keep us from attaining the full economic and environmental benefits of V2G (Thompson and Perez 2020 ) and that regulations are lagging behind technological developments (Freitas Gomes et al 2020 ). The lack of defined business models is seen by many experts as a key impediment (Noel et al 2019b ).
Major challenges that are linked to data-related aspects of VGI, including who has the right to access data from EVs (e.g. the state of EV batteries and charging) and how these data can be exploited, remain. Privacy concerns are one of the major obstacles to user acceptance (as is fear of loss of control over charging) (Bailey and Axsen 2015 ). In addition, there are also questions linked to cybersecurity (Noel et al 2019a ).
Nevertheless, VGI offers many opportunities that justify the efforts required to overcome these challenges. In addition to its services to the power system, VGI offers interesting perspectives for the full exploitation of synergies between EVs and renewable energy sources as both technologies promise large-scale deployment in the future (Kempton and Tomić 2005 , Lund and Kempton 2008 ). Exploiting EV batteries for VGI also is appealing from a life-cycle perspective, as the manufacturing of EV batteries has a non-trivial environmental footprint (Hall and Lutsey 2018 ). However, there are a few future developments that might compromise the potential of VGI, most notably cheaper batteries (including second-life EV batteries) that might compete with EVs for many potential services (Noel et al 2019b ). In addition, the impacts of new mobility business models, such as the rise of vehicle- and ride-sharing, on grid services remain unclear. Although smart charging will come first in the path toward grid integration, V2G services have the potential to provide additional value (Thingvad et al 2016 ).
7. Life-cycle cost and emissions
EVs differ from conventional ICEVs on an emissions basis. While the operation of gasoline- or diesel-powered ICEVs produces GHG and pollutant emissions that are discharged from the vehicle tailpipe, EVs have no tailpipe emissions. In a broader context, EVs still can be associated with so-called 'upstream' emissions from the processes that generate, transmit, and distribute the electricity that is used for their charging. Fueling an ICEV also involves upstream 'fuel-cycle' emissions from the raw-material extraction and transportation, refining, and final-product-delivery processes that make gasoline or diesel fuel available at a retail pump. These fuel-cycle emissions give rise to the colloquial jargon 'well-to-pump' emissions. Accordingly, a 'well-to-wheels' (WTW) life-cycle analysis (LCA) is an appropriate framework for comparing EV and ICEV emissions. WTW considers both upstream emissions from the fuel cycle ('well-to-pump') and direct emissions from vehicle operation ('pump-to-wheels') for a standardized functional unit and temporal period. WTW studies have a history of over three decades of use to evaluate direct and indirect emissions related to fuel production and vehicle operations (Wang 1996 ). WTW emissions are expressed typically on a per-mile or per-kilometer basis over a vehicle's assumed lifetime.
WTW analyses typically focus only on fuel production and vehicle operation. Some studies consider broader system boundaries that include vehicle production and decommissioning (i.e. recycling and scrappage) in an LCA framework. This broader system boundary considers what is commonly called the 'vehicle cycle' and provides a so-called 'cradle-to-grave' (or 'C2G') analysis. Vehicle-cycle emissions typically account for 5%–20% of today's ICEV C2G emissions and can be as low as 15% or as high as 80% of today's BEV emissions, depending on the underlying electricity-generation mix. Lower-carbon mixes result in vehicle-cycle emissions accounting for a greater portion of total emissions. As an extreme illustrative example, the case of zero-carbon electricity implies that vehicle-cycle emissions account for 100% of C2G emissions. In general, BEV vehicle-cycle emissions are 25% to 100% higher than their ICEV counterpart (Samaras and Meisterling 2008 , Ambrose and Kendall 2016 , Elgowainy et al 2016 , Hall and Lutsey 2018 , Ricardo 2020 ). As this section explores, higher initial BEV vehicle-cycle emissions almost always are counterbalanced by lower emissions during vehicle operation (with notable exceptions in cases in which BEVs are charged from especially high-emissions electricity).
Even including upstream emissions, EVs are championed as a critical technology for decarbonizing transportation (in line with anticipated widespread grid decarbonization). National Research Council ( 2013 ) identifies EVs as one of several technologies that could put U.S. on a path to reducing transportation-sector GHG emissions to 80% below 2005 levels in 2050. Furthermore, National Research Council ( 2013 ) estimates that BEVs would reduce emissions by 53%–72% compared to ICEVs in 2030. IEA ( 2019 ) contends, similarly, that EVs can reduce WTW GHG emissions by half versus equivalent ICEVs in 2030. Recently published literature also agrees, even on a C2G basis, estimating that future EV pathways offer 70%–90% lower GHG emissions compared to today's ICEVs (Elgowainy et al 2018 ). As such, the broad view across national, international, and academic-research perspectives is that EVs offer the potential to reduce transportation-related GHG emissions by 53% to 90% in the future.
Several studies find that EVs already reduce WTW GHG emissions today by as little as 10% or as much as 41% on average versus comparable ICEVs based on current electricity-production mixes. Samaras and Meisterling ( 2008 ), who are among the first to relate a range of potential electricity carbon intensities to associated EV-lifecycle emissions explicitly, estimate a 38%–41% GHG emissions benefit for EVs powered by the average 2008 U.S. grid. Hawkins et al ( 2012a ), informed by a meta-study of 51 previous LCAs, highlight great variations based on different electricity generation assumptions and vehicle lifetime. Hawkins et al ( 2012b ) estimate a decline of 10%–24% global warming potential (a measure proportional to GHG emissions) for EVs powered by the average 2012 European electricity mix. Elgowainy et al 2016 , 2018 ) estimate that EVs emit 20%–35% fewer GHG emissions when operating on the average 2014 U.S. grid mix.
Many factors contribute to variability in EV WTW emissions and estimated reduction opportunities compared to ICEVs—electricity-carbon intensity, charging patterns, vehicle characteristics, and even local climate (Noshadravan et al 2015 , Requia et al 2018 ). To illustrate these variabilities, figure 7 compares WTW GHG emissions of EVs versus comparable ICEVs. Relative emissions reductions are generally larger for larger vehicles. Woo et al ( 2017 ) find that electrifying SUVs reduces emissions more than electrifying sub-compact vehicles on a WTW basis versus comparable ICEVs (30%–45% and 10%–20%, respectively, assuming median national grid mixes). Ellingsen et al ( 2016 ) find that large EVs emit proportionally less than small EVs compared to comparable ICEVs on a C2G basis (27% and 19%, respectively).
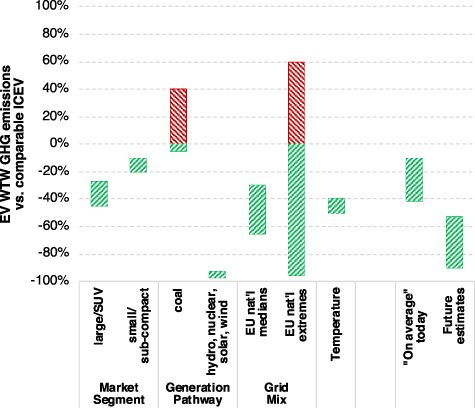
Figure 7. WTW GHG emissions for EVs versus comparable ICEVs on average and with illustrative variability by market segment, electricity generation pathway, grid mix, and ambient temperature.
Low-carbon electricity can lead to greater reductions in EV emissions. Electricity that is produced from coal, which has a high carbon intensity, can increase EV emissions by as much as 40% or decrease EV emissions by as much as 5% compared to an ICEV (depending on other assumptions). Conversely, electricity from hydropower, nuclear, solar, or wind, all of which offer near-zero carbon intensities, can decrease EV emissions by more than 95% compared to an ICEV (Woo et al 2017 ). Such variability in electricity-generation pathways affects the relative benefits of real-world grid mixes. For example, while EVs offer 30%–65% lower emissions versus comparable ICEVs on average in Europe (Woo et al 2017 , Moro and Lonza 2018 ), in individual countries relative emissions can range from as much as 95% lower to 60% higher (Orsi et al 2016 , Moro and Lonza 2018 ). Typically, U.S. EVs provide emissions reductions, but in some regions EV emissions are higher compared to an efficient ICEV (Reichmuth 2020 ). Changes in regional climate and daily weather add further variability: EV emissions can vary between 40% and 50% lower than a comparable ICEV even when charged from the same grid mix (Yuksel et al 2016 ). While outside the scope of a typical WTW comparison, the additional consideration of refueling infrastructure (i.e. gasoline stations for ICEVs and recharging equipment for EVs) is estimated to increase EV emissions by 4%–8% compared to a more modest 0.3%–0.7% increase for ICEV emissions (Lucas et al 2012 ).
When assessing EV emissions, average or marginal grid-emission factors are considered (Anair and Mahmassani 2012 , Traut et al 2013 , EPRI 2015 , Nealer and Hendrickson 2015 , Nealer et al 2015 , Elgowainy et al 2018 ), leading to significantly different results. Average emissions factors consider all electricity loads as equivalent, while marginal emission factors consider EVs as an additional load on top of existing electricity demands and estimate the associated incremental generation emissions. Marginal emissions could be higher or lower than average, depending on the relative emissions of marginal plants compared to the average in different regions. Different questions lead to using average or marginal metrics. Proper assessment of indirect EV emissions associated with electricity generation is complicated by numerous factors, including timescale (short or long term, aggregate or temporally explicit), system boundaries, impact of EV loads on power-system-expansion and -operation decisions, and non-trivial supply-demand synergies and allocation complexities. Yang ( 2013 ) reviews different grid-emissions-allocation methods concluding that there is no ideal approach to the allocation of emissions to specific end-use and stressing how different assumptions make it difficult to determine EV emissions and compare them to other alternatives and across studies. Nealer and Hendrickson ( 2015 ) discuss whether it is more appropriate to use marginal or average grid-emission factors to estimate EV emissions, concluding that 'average emissions may be the most accessible for long-term comparisons given the assumptions that must be made about the future of the electricity grid.'
Just as EVs offer typically a WTW-emissions reduction compared to ICEVs while shifting those emissions from the tailpipe to upstream, EVs shift costs as well. Operational (fuel and maintenance) costs of EVs are typically lower than those of ICEVs, largely because EVs are more efficient than ICEVs and have fewer moving parts. While data are still scarce, a recent Consumer Reports study estimates that maintenance and repair costs for EVs are about half over the life of the vehicle and that a typical EV owner who does most fueling at home can expect to save an average of $800 to $1000 a year on fuel costs over an equivalent ICEV (Harto 2020 ). Insideevs ( 2018 ) estimates a saving of 23% in servicing costs over the first 3 years and 60 000 miles (96 561 km). Borlaug et al ( 2020 ) estimate fuel savings between $3000 and $10 500 compared with gasoline vehicles (over a 15 year time horizon). However, vehicle capital costs for EVs are higher (principally due to the relatively high cost of EV batteries). In general, studies use a TCO metric to combine and compare initial capital costs with operational costs over a vehicle's lifetime. While some studies find that EVs are typically cost-competitive with ICEVs (Weldon et al 2018 ), others find that EVs are still more costly, even on a TCO basis (Breetz and Salon 2018 , Elgowainy et al 2018 ), or that the relative cost depends on other contextual factors, such as vehicle lifetime and use, economic assumptions, and projected fuel prices. Longer travel distance and smaller vehicle sizes favor relatively lower EV TCO (Wu et al 2015 ), as do lower relative electricity-versus-gasoline price differentials (Lévay et al 2017 ). Despite these differences regarding TCO conclusions across studies, there is general agreement that future EV costs will decline (Dumortier et al 2015 , Wu et al 2015 , Elgowainy et al 2018 ).
The existing literature suggests future EV emissions will decline, in large part due to expectations for continued grid decarbonization (Elgowainy et al 2016 , 2018 , Woo et al 2017 , Cox et al 2018 ). For example, Ambrose et al ( 2020 ) anticipate that evolution in vehicle types and designs could accelerate future decreases for EV GHG emissions. Several studies also posit repurposing used EV batteries for stationary applications could accrue additional GHG benefits (Ahmadi et al 2014 , 2017 , Olsson et al 2018 , Kamath et al 2020 ). Cox et al ( 2018 ) suggest future connectivity and automation technologies will enable energy-optimized EV-recharging behavior and associated lower carbon emissions. Similarly, future EV costs also are expected to decline as battery costs continue to decline (cf section 4 ), and new mobility modes such as ride-hailing lead to higher vehicle use that favors the business case for highly efficient EVs compared to ICEVs.
8. Synergies with other technologies, macro trends, and future expectations
Vehicle electrification fits within broader electrification trends, including power-system decarbonization and mobility changes. The latter include micro-mobility in urban areas, new mobility business models revolving around 'shared' services as opposed to vehicle ownership (e.g. ride-hailing and car-sharing), ride pooling, and automation. These trends are driven partially by the larger availability of efficient and cost-effective electrified technologies (Mai et al 2018 ) and the prospect of abundant and affordable renewable electricity and by other technological and behavioral changes (e.g. real-time communication). Abundant and affordable renewable electricity is a conditio sine qua non for EVs to provide a pathway to decarbonize road transportation. Direct use of PV on-board vehicles (i.e. PV-powered or solar vehicles) also is being considered. However, this concept still faces many challenges (Rizzo 2010 , Aghaei et al 2020 ). Yamaguchi et al ( 2020 ) show potential synergies for integration but also highlight that for this technology to be successful, the development of high-efficiency (>30%), low‐cost, and flexible PV modules is essential.
Urban micro-mobility is emerging recently as an alternative to traditional mobility modes providing consumers in most metropolitan areas worldwide with convenient options for last-mile transportation (Clewlow 2019 , Zarif et al 2019 , Tuncer and Brown 2020 ). Virtually all micro-mobility solutions use all-electric powertrains. Shared electric scooters and bikes (often dockless), e.g. those pioneered by Lime and Bird in the U.S., are experiencing rapid success and are 'the fastest-ever U.S. companies to reach billion-USD valuations, with each achieving this milestone within a year of inception' (Ajao 2019 ). Future expectations for micro-mobility remain uncertain due to issues related to sidewalk congestion, safety, and vandalism (heavily impacting the business case for these technologies). However, the nexus with EVs has not been questioned.
Similarly, ride-hailing—matching drivers with passengers at short notice for one-off rides through a smartphone application, which date back to Uber's introducing the concept in 2009—is an attractive alternative to traditional transportation solutions. These mobility-as-a-service solutions cater to the consumer's need for quick, convenient, and cost-effective transportation and may lead to drops in car-ownership and driver-licensure rates (Garikapati et al 2016 , Clewlow and Mishra 2017 , Movmi 2018 , Walmsley 2018 , Henao and Marshall 2019 , Arevalo 2020 ). After just over 10 years, ride-hailing is widely available and extremely successful, with hundreds of millions of consumers worldwide and 36% of U.S. consumers having used ride-hailing services (Mazareanu 2019 ). While most ride-hailing vehicles today are ICEVs (in line with the existing LDV stock), many ride-hailing companies are exploring electrification opportunities (Slowik et al 2019 ). EVs offer a number of potential advantages as high vehicle usage promotes a more favorable business model for recovering the higher EV purchase price by leveraging cheaper fuel costs (Borlaug et al 2020 ). At the same time, long-range vehicles and effective charging solutions are required for ride-hailing companies to transition to EVs (Tu et al 2019 ). Moreover, EVs can mitigate additional fuel use and emissions related to increased travel, mostly due to deadheading, which is estimated to be ∼85% (Henao and Marshall 2019 ). EVs also provide access to restricted areas in some cities (driving some regional goals for ride-hailing electrification). For example, Uber aims for half of its London fleet to be electric by 2021 and 100% electric by 2025 (Slowik et al 2019 ).
Automation trends are also poised to have the potential to disrupt transportation as we know it. The combination of electric and connected automated vehicles (CAVs) is hypothesized to offer natural synergies, including easier integration with CAV sensors and a greater affinity for cheaper fuels aligning with greater travel (Sperling 2018 ). The chief counterargument relates to high power requirements for a heavily instrumented CAV, which would deplete EV batteries quickly and may be accommodated better with PHEV powertrains. Wireless EV charging, both stationary and dynamic, increases the potential synergies enabling autonomous recharging. Also, CAVs may be required to maximize the efficiency of dynamic wireless charging. In fact, without the alignment accuracy enabled by CAVs, in-road dynamic charging may have limited efficacy. The literature on these synergies is relatively sparse, though some studies are beginning to investigate the implications of combining EV and CAV technologies.
Even though the technology is not widely available commercially, several studies are beginning to examine how consumer preferences may be influenced by the combination of connected, automated, and electric vehicles 15 . Thiel et al ( 2020 ), for example, highlight how full EV success may emerge as automated shared vehicles become predominant in a world where the border between public and private transport will cease to exist. Tsouros and Polydoropoulou ( 2020 ) develop a survey combining traditional attributes (e.g. car type and vehicle style) alongside future technology attributes (e.g. fuel type and degree of automation) and estimate preferences using a latent-class structural-regression approach. They find a specific class of consumers, described as technology-savvy, who have a high proclivity for both alternative-fuel vehicle technologies and higher degrees of automation. While the proportion of the population that can be classified as technology-savvy is unclear, Tsouros and Polydoropoulou ( 2020 ) provide early compelling evidence that consumers see explicit value in the combination of EVs and automation. Hardman et al ( 2019 ) provide a complementary perspective of early adopters of automated vehicles based on a survey of existing U.S. EV owners. Similar to the work of Tsouros and Polydoropoulou ( 2020 ), Hardman et al ( 2019 ) find that the type of consumers who would pursue automated vehicles have similar lifestyles, attitudes, and socio-demographic profiles as EV adopters. These include high-income consumers, with high levels of knowledge about technology features, who have positive perceptions of CAV attributes and technology in general, provided that safety concerns are resolved.
Another benefit of the combined technologies is the potential to integrate charging events better with the needs of the electricity grid. Several studies assess the combination of these technologies with new mobility services such as car-sharing systems to optimize VGI. Iacobucci et al ( 2018 ) consider a case study in Tokyo of the ability of connected, automated EVs to be dispatched to respond to both transportation demand and charging to meet demands and constraints of the electricity system. The authors observe the vehicles can take advantage of a variety of different time-of-day pricing structures—leading to a tradeoff between wait times and cost benefits from lower fuel prices. They find that the vehicles in Tokyo can supply on the order of 3.5 MW of charging flexibility per 1000 vehicles, even during times of high mobility demand. Miao et al ( 2019 ) conduct a similar study in a generic region. The authors develop an algorithm that simulates operational behavior of the connected, automated EV technology that includes trip demand and vehicle usage, vehicle relocation, and vehicle charging. Their results indicate that charging behavior is highly sensitive to different levels of charging due to the length of charging—which can affect service provision of trip demand.
The final topic of study considering synergistic opportunities between connected, automated vehicles and EVs focused on emissions benefits. Taiebat et al 2018 explore the environmental impacts of automated vehicles showing net positive environmental impacts at the local vehicle-urban levels due to improved efficiency, but acknowledge that greater vehicle utilization and shifts in travel patterns might to offset some of these benefits. Of course, EVs provide the significant benefit of eliminating tailpipe criteria-pollutant emissions, yielding significant human-health benefits. Regarding GHG emissions, two of the earliest studies on this topic examine the net effect of automation on reducing transport GHG emissions (Brown et al 2014 , Wadud et al 2016 ). Greenblatt and Saxena ( 2015 ) conduct a case-study application of connected and automated vehicles in taxi fleets and find large emissions benefits associated with electrification. They find a decrease of GHG emissions intensities ranging from 87% to 94% below comparable ICEVs in 2014 and 63% to 82% below hybrid electric vehicles (HEVs) in 2030. The total emissions benefit is augmented relative to privately owned vehicles due to the higher travel intensity of taxi vehicles. Following these earlier works, additional case studies examine the hypothetical application of automated and electric fleets. These include two studies in Austin, Texas. Loeb and Kockelman ( 2019 ) examine a variety of scenarios to simulate the operation of different vehicle fleets replacing current-day transportation network companies and taxis. The primary goal of their work is to estimate costs associated with operation. They find that automated EVs are the most profitable and provide the best service among the vehicle-technology options that they examine. Gawron et al ( 2019 ) also perform a case study in Austin, Texas, but focus on the emissions benefits of electrifying an automated taxi fleet. They find that nearly 60% of emissions and energy in a base case CAV fleet can be reduced by electrifying powertrains. These improvements can be pushed up to 87% when coupled with grid decarbonization, dynamic ride-sharing, and various system- and technology-efficiency improvements. These results are consistent with a more generalized study by Stogios et al ( 2019 ), who, in a similar approach simulating fleet behavior, find that emissions from CAVs are most dramatically improved via electrification.
While EVs are a relatively new technology and automated vehicles are not widely available commercially, the implications and potential synergies of electrification and automation operating in conjunction are significant. The studies mentioned in this section are investigating a broad set of impacts when CAVs are coupled with EVs. Future research is necessary to generalize and refine many of these results. However, the potential for transformative changes to transportation emissions is clear.
8.1. Expectations for the future
EVs hold great promise to replace ICEVs for a number of on-road applications. EVs can provide a number of benefits, including addressing reliance on petroleum, improving local air quality, reducing GHG emissions, and improving driving experience. Vehicle electrification aligns with broader electrification and decarbonization trends and integrates synergistically with mobility changes, including urban micro-mobility, automation, and mobility-as-a-service solutions. The effective integration of EVs into power systems presents numerous opportunities for synergistic improvement of the efficiency and economics of electromobility and electric power systems, with EVs capable of supporting power-system planning and operations in several ways. Full exploitation of the synergies between EVs and VRE sources offers a path toward affordable and clean energy and mobility for all, as both technologies promise large-scale deployment in the future. To enable such a future continued technology progress, investments in charging infrastructure (and related building codes), consumer education, effective and secure VGI programs, and regulatory and business models supporting all aspects of vehicle electrification are all critical elements.
The coronavirus pandemic is impacting LDV sales in most countries negatively, and 2020 EV sales are expected to be lower than 2019, marking the first decline in a decade (BloombergNEF 2020 ). However, sales of ICEVs are set to drop even faster and, despite the crisis, EV sales could reach a record share of the overall LDV market in 2020 (Gul et al 2020 ). Despite these short-term setbacks, long-term prospects for EVs remain undiminished (BloombergNEF 2020 ).
Several studies project major roles for EVs in the future, which is reflected in massive investment in vehicle development and commercialization, charging infrastructure, and further technology improvement, especially in batteries and their supply chains. Consumer adoption and acceptance and technology progress form a virtuous self-reinforcing circle of technology-component improvements and cost reductions that can enable widespread adoption. Forecasting the future, including technology adoption, remains a daunting task. Nevertheless, this detailed review paints a positive picture for the future of EVs for on-road transport. The authors remain hopeful that technology, regulatory, societal, behavioral, and business-model barriers can be addressed over time to support a transition toward cleaner, more efficient, and affordable mobility solutions for all.
Acknowledgments
The authors thank Paul Denholm, Elaine Hale, Trieu Mai, Caitlin, Murphy, Bryan Palmintier, and Dan Steinberg for valuable comments on figure 6 , as well as two anonymous reviewers for helpful comments on the paper. This work was co-authored by National Renewable Energy Laboratory (NREL), which is operated by Alliance for Sustainable Energy, LLC, for U.S. Department of Energy (DOE) under Contract No. DE-AC36-08GO28308. No funding was received to support this work. The views expressed in this article do not necessarily represent the views of DOE or the U.S. Government. The findings and conclusions in this publication are those of the authors alone and should not be construed to represent any official U.S. Government determination or policy, or the views of any of the institutions associated with this study's authors.
EVs are defined as vehicles that are powered with an on-board battery that can be charged from an external source of electricity. This definition includes plug-in hybrid electric vehicles (PHEVs) and battery electric vehicles (BEVs). EVs often are referred to as plug-in electric vehicles (PEVs).
Transport electrification is confined not only to electric LDVs. Transport electrification includes a wide range of other vehicles, spanning from small vehicles that are used for urban mobility, such as three-wheelers, mopeds, kick-scooters, and e-bikes, to large urban buses and delivery vehicles. In 2019, the number of electric two-wheelers on the road exceeded 300 million and buses approached 0.6 million (IEA 2019 , Business Wire 2020 ), with new deliveries in 2019 close to 100 thousand units (EV Volumes 2020 ).
Just over 10% of the U.S. heavy-duty truck (Class 7–8) population requires an operating range of 500 miles (805 km) or more, while nearly 80% operate within a 200 mile (322 km) range and around 70% within 100 miles (161 km). Only ∼25% of heavy truck VMT require an operating range of over 500 miles (805 km) (Borlaug et al Forthcoming ).
As a counterargument, Tesla states that 'all new Tesla cars come standard with advanced hardware capable of providing Autopilot features today, and full self-driving capabilities in the future—through software updates designed to improve functionality over time'.
A Literature Review on Electric Vehicles: Architecture, Electrical Machines for Power Train, Converter Topologies and Control Techniques
Ieee account.
- Change Username/Password
- Update Address
Purchase Details
- Payment Options
- Order History
- View Purchased Documents
Profile Information
- Communications Preferences
- Profession and Education
- Technical Interests
- US & Canada: +1 800 678 4333
- Worldwide: +1 732 981 0060
- Contact & Support
- About IEEE Xplore
- Accessibility
- Terms of Use
- Nondiscrimination Policy
- Privacy & Opting Out of Cookies
A not-for-profit organization, IEEE is the world's largest technical professional organization dedicated to advancing technology for the benefit of humanity. © Copyright 2024 IEEE - All rights reserved. Use of this web site signifies your agreement to the terms and conditions.
- Open access
- Published: 15 December 2022
Integration of EVs into the smart grid: a systematic literature review
- Vivian Sultan 1 ,
- Arun Aryal 1 ,
- Hao Chang 1 &
- Jiri Kral 1
Energy Informatics volume 5 , Article number: 65 ( 2022 ) Cite this article
7568 Accesses
10 Citations
Metrics details
Integration of electric vehicles (EVs) into the smart grid has attracted considerable interest from researchers, governments, and private companies alike. Such integration may bring problems if not conducted well, but EVs can be also used by utilities and other industry stakeholders to enable the smart grid. This paper presents a systematic literature review of the topic and offer a research framework to guide future research and enrich the body of knowledge. The systematic literature review presented in this paper does not contain all the material available on this subject. It does, however, include most of the key publications readily available in a power-utility or technical-reference library together with some of the earlier papers in the field (the anchor papers). For this review, we selected appropriate digital sources (digital libraries and indexing systems; IEEE Xplore and Web of Science), determined the search terms, and conducted a broad automated search. This article also details the components of the research theme—EV integration into the smart grid—as well as its accompanying use cases. The analysis of the relevant papers indicated four types of key research concerns: power-grid, power-system, and smart-grid reliability and the impacts of changes on them. These results can help guide future research to further smart-grid development. Future research can also expand the reach of this research to address its limitations in scope and depth.
Introduction and research background
Integration of electric vehicles (EVs) into the smart grid can be leveraged by utilities and other industry stakeholders to bring several benefits and to enable the smart grid. Mwasilu et al. ( 2014 ) emphasized the importance of vehicle to grid (V2G), an example of services on the grid that will allow the shift of the static power system to the efficient virtual power grid. San Diego Gas and Electric ( 2015 ) reported that deploying networks of EV charging stations can stabilize and bring advantages to the grid in locations with excess power; EV charging can absorb mid-day solar overgeneration and alleviate wind curtailment at night. Sultan et al. ( 2017 ) highlighted how charging EVs when nondispatchable assets, such as solar and wind generators, are producing more energy can help flatten out the demand curve and reduce the extent to which supply suddenly escalates. All these characteristics reduce system costs, benefit ratepayers, and improve the profitability of generators (San Diego Gas and Electric 2015 ).
On the international stage, the EV industry has been prominently used as a tool by countries to meet their carbon-footprint-reduction goals. EVs also have spurred a potential new avenue of electricity sales while at the same time impacting maintenance costs by adding to peak loads and changing historical grid-load patterns. The integration of EVs with electrical grids is giving rise to the concept of smart grids. This integration can come from potential bidirectional charging (V2G), grid storage research, and innovative energy generation (Denholm et al. 2015 ).
EVs can potentially serve a dual purpose, an alternate form of grid storage offloaded to the public. It can allow the vehicle owners to be compensated for providing electric service when the vehicle is not in use, helping to reduce the cost of ownership. Also, the quality of life for large urban centers can increase due to the potential opportunity to move emissions from large population centers. This relocation of energy production can improve air quality and public health in metropolitan cities, while remaining emission production further decreases as remaining energy needs are met by renewable resources (Denholm et al. 2015 ).
However, it is important to note that the integration of many EVs into the electric power system is a major challenge which requires a thorough evaluation and examination in terms of economic impacts, operation, and control benefits at ideal circumstances (Mwasilu et al. 2014 ). Large-scale integration of EVs into the smart grid may bring a series of problems if EVs are not integrated carefully into the smart grid. According to Green et al. ( 2011 ), several works analyzed the impact of EVs on the power-distribution system. Examples of the anticipated adverse impacts are power transformers overheating and the need for new investments in distribution facilities (Mwasilu et al. 2014 ).
A major challenge is the impact of simultaneously charging many EV batteries on the power network, this could change the overall load profile of the grid significantly. The issue is that the charging behaviors of EVs are only regulated by the customer so it’s not within the control of the grid operators and the electric utilities. The risk of grid overload can lead to a degradation of the grid performance, bad power quality and/or voltage deviations, even a blackout of the whole power system if EV charging is not managed properly. However, time-of-use rates can guide an EV charging, and V2G benefits can be easily ramped up and down in response to the load on the system, improving voltage regulation and droop control.
The high cost of integration due to inadequate charging infrastructure and competition from other energy-storage technologies are additional challenges to EV integration. Pumped hydroelectric storage is considered, for example, to be much cheaper than the V2G option. According to Hernández-Moro et al. ( 2012 ) and Mullan et al. ( 2012 ), pumped hydro has greater efficiency (up to 99%) and can store energy for long periods as compared to the EV battery (Hernández-Moro et al. 2012 ).
On the other hand, EVs have advantages when operated in the V2G mode to feed power to the utility grid. The primary advantages stem from the EV battery’s ability to provide power when needed. EV technology can provide grid support by delivering ancillary services such as peak power shaving, spinning reserve, and voltage and frequency regulation (Ehsani et al. 2012 ).
Peak shaving means reducing the highest demand levels at the power plant. From a utility perspective, EVs can be viewed as both dynamic loads which may not be easy to predict, but also potential backup for the electric grid through the V2G technology (Mwasilu et al. 2014 ). So, EVs can respond to changes in demand, they provide a spinning reserve and can dramatically reduce the need to use expensive peaking plants, savings that utilities can pass on to their customers in the form of lower energy costs.
Another point is that mismatches between power supply and demand can lead to oscillations in the supplied voltage, phase angle, and frequency. These oscillations degrade power quality, possibly damaging utility customers’ sensitive electronic equipment. EVs can both provide and absorb power and energy so to help dampen both intra- and interarea power oscillations. Mwasilu et al. ( 2014 ) claim that EVs have the potential to assist in voltage and frequency regulation, thus enhancing electric-grid reliability and power quality.
A modern grid that is well equipped to handle the additional EV loads and reap the benefits from the intersection of the EVs and the electric power network could contribute to an enhanced grid with real environmental benefits for all customers.
Thus, more research is needed, and it is critical especially with the increasing penetration of EV charging into the electric power system. Unease about global warming, energy security, and the current health of the environment has caused more interest in EVs. The existing power grid suffers from unpredictable and intermittent supply of the electricity from wind and photovoltaic (PV) solar sources, so EV charging and V2G services are a promising solution to balance the generation from renewable energy sources (International Electrotechnical Commission 2012 ).
The intent of this paper is to present a systematic literature review of EV integration into the smart grid and develop a research framework to guide future research and enrich the body of knowledge. A systematic literature review is a particularly influential tool in research; it allows a scholar to gather and recap all the information about a specific field (Spanos and Angelis 2016 ).
This paper is organized as follows. In the “Introduction and research background”, we introduce and present the topic’s research background. In the “Methodology”, we delineated our research methodology. The “Results” describes the results of the review and offers a bibliography of anchor papers. The “Discussion” discusses the results. Finally, “Conclusion” concludes.
Methodology
This paper’s systematic literature review follows the three stages defined by Kitchenham ( 2004 ) and Kitchenham et al. ( 2009 ): planning, conducting, and reporting. In the first stage, we create the research protocol. The second stage is the actions of reviewing literature based on the protocol, and the last stage manifests the article’s results section.
Research protocol
The research protocol established in the planning stage guided the systematic literature review. The first step is to identify the need for a systematic review. Although several studies try to investigate EV integration into the smart grid, no comprehensive review has so far summarized all these studies and offered a deeper insight. Therefore, the need for a systematic literature review providing solid foundations and equipping researchers with pertinent information is clear.
To develop the review protocol, we define the research questions, select the search strategy, establish the study’s inclusion and exclusion criteria, select quality-assessment criteria, and identify the data to be extracted from the studies. Three research questions guide our review.
What are the themes within research on EV integration into the smart grid?
What are the results of the studies?
What research methods are used?
To conduct the systematic literature review, we adopted a broad automated search, a method that includes the selection of the most appropriate digital sources (digital libraries and indexing systems) and the determination of the search terms (Spanos and Angelis 2016 ). We selected two digital libraries, IEEE Xplore and Web of Science, as they are the most relevant digital sources in electricity-infrastructure research. Our searches relied on the titles of the papers to avoid retrieving irrelevant papers. The search strings we used were.
Inclusion and exclusion criteria
According to Spanos and Angelis ( 2016 ), a systematic literature review’s inclusion and exclusion criteria must be distinct and clearly stated. We used three selection criteria and one exclusion criterion in our systematic review.
Quality assessments
Once each paper has passed the relevancy test, we peruse the papers based on the quality-assessment criteria. These rigorous criteria ensure all studies included in the systematic literature review achieve an adequate level of quality. After consulting with domain experts and independent researchers, we decided to include each article for the final analysis if it satisfies three criteria:
The data description is available, and its existence can be verified.
Research methodology is clearly described.
Results presentation is clear and impactful.
Once the articles had passed the inclusion and quality-assessment criteria, we recorded the data features of the papers for the final analysis: (1) title, (2) authors, (3) publication year, (4) authors’ affiliations, (5) journal title, (6) number of papers citing the article, (7) abstract, (8) research questions, (9) research methodology, and (10) results.
For this survey, we analyzed results from 724 papers relevant to integration of EVs into the smart grid, 260 papers from the IEEE Xplore and 464 papers from the Web of Science. The analysis process considered the research results, as stated by the authors, and was mainly conducted throughout the abstract, conclusion, and results sections if required. Tables 1 and 2 present counts of document identifiers and publication years. Table 3 presents summary statistics.
The analysis of the relevant papers indicated four types of key research concerns.
• Assessing power-grid reliability considering EV integration.
• Improving power-system reliability considering EV integration.
• Planning for smart-grid reliability in preparation for EV integration.
• Evaluating the impacts of adding EVs and charging stations on grid reliability.
The research methodologies of the included papers can be classified into two categories, analytical and simulation. Analytical techniques “represent the system by mathematical models and evaluate the reliability indices from these models using mathematical solutions” (Faulin et al. 2010 ). Simulation views problems as a series of real experiments, such as Monte Carlo simulation which is used for the prediction of probability for various outcomes when dealing with random variables. Table 4 presents the statistics for the different research methods. Finally, 58 papers used the Monte Carlo simulation method.
Based on the results of the systematic literature review, we have constructed a smart-grid–EV-integration framework highlighting research components of EV integration into the smart grid, with goals, themes, use cases, and research tools.
Research contribution: framework
In this section, we propose the smart-grid–EV-integration framework with three main research domains (Fig. 1 ), solutions for charging, microgrids and distributed generation, and managing power demand. This framework recognizes these three EV-integration domains as cardinal research-movement drivers for studying the benefits and challenges of integrating EVs into a smart grid. We also propose technological and socioeconomic solutions. Next, based on our literature analysis, we present common themes within the domains. The first theme, integrating EVs, drives the enhancement of EV technologies. The second theme, advancement of the smart grid, drives the advancement of distributed generation. The third theme, smart-grid reliability with EV applications, drives power-demand management and smart-grid reliability in the context of EV integration.
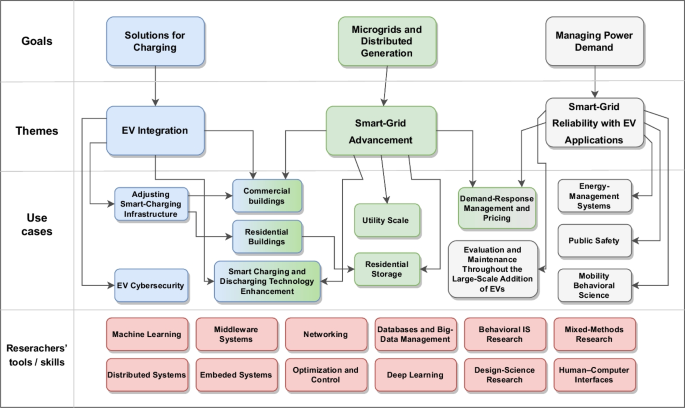
Smart-Grid–EV integration framework
The use cases in the research framework are classified based on under which research theme(s) they fall (see Fig. 1 ). Smart-charging infrastructure use cases deal with the reformation of current structures to achieve both physically and technically suitable charging solutions. “Apart from the technological innovation of EV, effective charging infrastructure plays a fundamental role in supporting the wider adoption of EV” (Chen et al. 2020 ). An important area of infrastructure adjustments is, for instance, planning for EV smart charging stations, involving both geographical research and charging-management system implementation.
According to Zhou et al. ( 2021 ), commercial buildings with EV charging stations and PV panels are common prosumers in the smart grid. Thus, “the energy management of commercial buildings has significant potential for electricity cost saving, load levelling, and distributed generation consumption.” Site-integrated EV charging solutions for workplaces and other business or commercial areas are interesting use cases, where growth in EV smart-grid penetration calls for improved charging load management. Algorithms, charging and discharging impacts on smart-grid peak loads, and cost benefits are worthy of investigation.
Residential-building use cases explore the impacts of EV integration on a household, very often in combination with implementation of photovoltaic or wind-energy appliances. Charging solutions can have economic benefits. Residential storage, on the other hand, like EVs, can be both flexible and time shiftable and can significantly increase residential-demand elasticity (Rassaei et al. 2015 ). The use cases explore how to manage smart-home energy in a residential smart grid and how energy stored in the EV can be used for distributed generation either for the household or for a larger residential area. This area also involves associated-risks investigation, including increased power losses, overloads, and voltage fluctuations, and how they influence the smart grid.
EV Cybersecurity use cases investigate EV integration into the smart grid from the perspectives of energy safety, usage, user information, and transactions. According to Sanghvi et al. ( 2021 ), EV integration can potentially leave the grid “vulnerable to cyberattacks from both legacy and new equipment and protocols, including extreme fast-charging infrastructure.” Since EV participation in the smart grid will unavoidably partially depend on accessing power and communication networks and systems, such a system might become a target of such attacks. Thus, cybersecurity and energy security are crucial areas of research around smart-grid–EV integration.
Technological enhancement of smart charging and discharging evaluates problems caused by advancements in charging technologies to identify strategies, benefits, and risks and evaluate and propose how EV charging and discharging can help improve a smart grid’s flexibility and effectiveness in response to energy fluctuations in the distribution area. According to Aghajan-Eshkevari et al. ( 2022 ), “it is essential to manage the charging and discharging of EVs [that] can be also considered sources of dispersed energy storage and used to increase the network’s operation efficiency with reasonable charge and discharge management.” Use cases may also include improvement of fast-charging technologies, battery technologies, wireless charging, and roadway electrification.
Utility-scale energy storage solutions help maintain a balance between energy generation and consumption in the smart grid. As the EV market grows, more degraded batteries can be further used for other purposes. “In particular, the repurposing of EV [lithium-ion batteries] in stationary applications is expected to provide cost-effective solutions for utility-scale energy storage applications” (Steckel et al. 2021 ). Use cases in this category involve addressing questions of battery recycling sustainability, degradation, participation of EVs in the load balancing through the dispatch of batteries, and other areas.
Demand-response management and pricing use cases focus on both technical and socioeconomic areas of energy supply and demand for smart-grid operators, charging-station operators, and EV users and their demand response during the peak time. While smart-grid–EV integration has a significant impact on energy demand, they also represent an energy resource. “Therefore, in smart grid, the consumer demand is expected to be controlled so as to coordinate with the electricity generation, which is the main objective of demand response management” (Yu et al. 2016 ). Power demand response can positively impact peak shaving and load balancing, and open new possibilities for energy market and energy trading through energy-aggregator demand-response programs (Ren et al. 2021 ).
Evaluation and maintenance throughout the addition of EVs can involve mathematical modeling for both technological and economic evaluation of EV deployment’s impacts on the smart grid, predictive maintenance solutions for distribution transformers under the increased EV load, energy-dispatch strategies, involved systems’ life cycles, charging behavior, and other methods to maintain a balanced smart grid with growing numbers of EVs.
Energy-management systems are crucial to the smart grid’s ecosystem. Integrated EVs can contribute to the important task of effectively maintaining the power supply–demand balance and decrease the peak load. Energy-management systems also handle sharing or exchanges among different energy sources, including EVs, to establish reliable and effective supply. Use cases in this category involve many topics like energy-quality management systems, optimization systems, energy-consumption control systems, and scheduling systems. Energy-management systems are also closely linked to demand management and response (Meliani et al. 2021 ).
Public safety in EV adoption must be considered. As countries around the globe strive to meet energy objectives while decreasing their climate impact, it is crucial to identify and regulate new EV-related technologies to protect the public from potential undesirable effects. A growing number of EVs increases risks from, for instance, disposed or damaged batteries. Therefore, proper risk-mitigation techniques—professional training, recycling policies, and standardization—must ensure public safety and environmental protection (Brown et al. 2010 ).
Mobility behavioral science explores the questions associated with human behavior’s impact on transportation and energy and is crucial for understanding of how future EV and smart-grid technologies should be implemented. According to Rames et al. ( 2021 ), “exploring multidimensional aspects of differences in technology adoption, travel, and vehicle ownership across settlement types can help inform energy-efficient and affordable mobility system goals.” Thus, research in this area involves modeling EV owners' usage and driving behavior for effective power planning and operations, drivers’ motivation to participate in peak shaving, and implementation of localization technologies to adjust charging behavior and manage demand.
We aim to help resolve problems in the EV-integration field using such tools as machine learning, deep learning, networking, distributed systems, middleware systems, embedded systems, optimization and control, databases and big data management, human–computer interfaces, behavioral information systems research, design-science research, and mixed-methods research. When applied to the EV-integration field, these tools have the potential to significantly increase knowledge in the field and solve some of the problems it faces.
Smart-grid–EV-integration research framework founded on the previous literature
In addition to our smart-grid–EV-integration perspective, Fig. 2 illustrates our smart-grid–EV-integration research framework founded on the previous literature and our view of the domain. The framework focuses on two main areas: EV integration and the smart grid. The first research focus involves systems connecting EVs, transportation infrastructure, power grid, buildings, and renewable energy sources (Meintz 2022 ). Adjusting infrastructure, charging solutions, and associated costs are all possible goals in the EV-integration research focus.
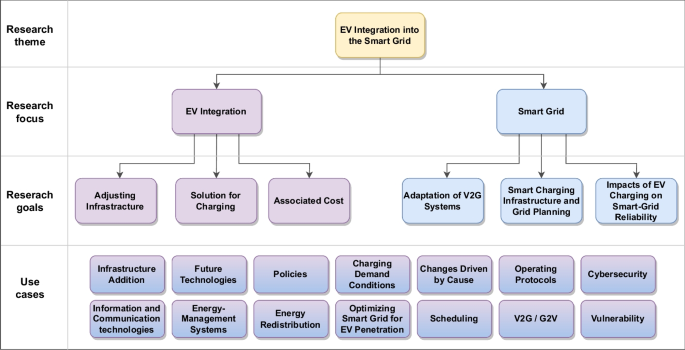
Smart-grid–EV-integration research framework
The smart grid (the second research focus) refers to the electric grid, a network of transmission lines, substations, transformers, and more that deliver electricity to a set location, integrated with digital technology allowing for sensing and two-way communication between the utility and its consumers (Department of Energy, Office of Electricity 2022 ). Adaptation of V2G systems in the smart grid, smart charging infrastructure, grid planning, and impacts of EV charging on the smart grid’s reliability are the possible goals within the smart-grid research focus.
In the context of a smart-grid–EV-integration research framework, scenarios and use cases can be classified into infrastructure addition, future technologies, policies, charging-demand conditions, changes driven by causes, operating protocols, cybersecurity, information and communication technologies, energy-management systems, energy redistribution, optimizing the smart grid for EV penetration, scheduling, V2G, and vulnerability.
Anchor papers on the integration of EVs into the smart grid
One way to ensure the grasp of the main core of a subject is to examine the references cited in the current articles and highlight repeatedly cited papers. In this literature review, papers cited more than one standard deviation above the average are considered anchor papers.
From the articles that passed the filter criteria, we identified anchor papers, highly cited and influential papers. To identify anchor papers, we used the same search string without an exclusion criterion (no year-range restriction) to pull all journal and magazine publications matching the search criteria from the database. We sorted the extracted articles (700 total) in descending order based on the number of articles citing each focal article, calculated the standard deviation for the articles’ number of citations, and identified the outliers (articles whose number of citations exceeded one standard deviation greater than the mean). The search string that we used for IEEE Xplore is ("Document Title":electric vehicle) AND ("Document Title":smart grid) and refined by Content Type: Conferences, Journals, and Early Access Articles. Meanwhile, for Web of Science, we used the search TOPIC: (electric vehicle AND smart grid) refined by DOCUMENT TYPES = (ARTICLE) Timespan = All years. Indexes = SCI-EXPANDED, SSCI, A&HCI, ESCI.
Based on this analysis, we found 61 anchor papers and passed them through a relevancy test to see if they are related to grid-reliability research. As each paper passed the relevancy test, we peruse it to apply quality-assessment criteria. After excluding the irrelevant papers and those not meeting the quality criteria, we identified 45 anchor papers (Table 5 ).
Table 6 presents a bibliography and analysis of anchor papers on the subject of the integration of EVs into the smart grid. Based on this analysis, Table 7 presents the distribution and percentages of anchor articles by research theme or question previously identified as follows. Optimizing grid usage to EV needs and the impacts on EV applications of smart-grid implementation or V2G communication have received the most attention. Environmental changes due to EV or grid applications show the lowest percentages of anchor articles.
Table 8 presents the research methods used by the authors of the anchor papers. Though simulation is the dominant research method considering the entire literature, articles using analytical approaches seem to get more attention based on articles citing them.
Bearing in mind the research themes and the methods illustrated in the anchor papers, we posit that simulation has been a popular topic in research and that there is need for more research in the area of environmental changes due to EV or grid applications, how to adjust the reliability and adequacy of charging stations and impacts on EV and grid applications.
Analytics using machine learning and big-data management would help the research community plan and improve EV integration into the smart grid. In future research, we intend to highlight the novel use of analytics to predict research themes such as environmental changes due to EV or grid applications, how to adjust the reliability and adequacy of charging stations and impacts on EV applications of grid applications. The goal is to offer an enhanced viewpoint for this research topic, while considering the impact of changes, EVs’ integration, and the potential benefits to the smart grid.
To enable more robust research on smart-grid–EV integration, we both plan our own research and invite other authors to submit original papers on topics including, but not limited to,
EV battery charging optimization
Mobility behavioral science
Location analytics for EV integration
Demand management and pricing for EVs in the electricity network
Internet of things and sensors for EV integration
Recent advancement in analytics for EV integration and microgrids
Innovative charging strategies for EVs
As described in the previous section, this systematic literature review provides a solid foundation to equip researchers with pertinent information.
The intent of this paper is to present a systematic literature review of smart-grid–EV integration and offer a research framework to guide future research and enrich the body of knowledge. Because the systematic literature review presented in this paper focuses on two digital libraries and searches only article titles, it does not contain all the material available on this subject. It does, however, include most of the key publications readily available in a power-utility or technical-reference library together with some of the earlier papers in the field (the anchor papers).
To conduct a systematic literature review, we completed a broad automated search, a method that includes the selection of appropriate digital sources (digital libraries and indexing systems) and the determination of the search terms. We selected the digital libraries IEEE Xplore and Web of Science for the systematic review. This article also details the components of our research theme and its accompanying use cases.
This review is limited to the IEEE Xplore and Web of Science digital libraries to facilitate an automated search of the literature. This limitation is reasonable because these sources are most likely to be available in a power-utility or technical-reference library. However, a broader search may find other research not analyzed here. Additionally, the search was restricted to article titles to reveal those research projects most closely related to the topics of interest. A broader search of abstracts and full text would certainly find more articles, but would likely involve considerably more false positives.
Analytics using machine learning and big-data management could help the research community plan and improve smart-gid–EV integration. Based on the four types of key research concerns (power-grid, power-system, and smart-grid reliability and the impacts of changes on them), we intend to highlight the novel use of analytics to predict research themes in future research. The goal is to offer an enhanced viewpoint for smart-grid–EV integration while considering the impact and the potential benefits to the smart grid of such changes and EVs’ integration.
Availability of data and materials
Additional supporting data is available from the first author.
Abbreviations
Electric vehicle
Photovoltaic
- Vehicle to grid
Aghajan-Eshkevari S, Azad S, Nazari-Heris M, Mohammad TA, Asadi S (2022) Charging and discharging of electric vehicles in power systems: an updated and detailed review of methods, control structures, objectives, and optimization methodologies. Sustainability 14(4):2137. https://doi.org/10.3390/su14042137
Article Google Scholar
Ahmadi L, Young SB, Fowler M, Fraser RA, Achachlouei MA (2017) A cascaded life cycle: reuse of electric vehicle lithium-ion battery packs in energy storage systems. Int J Life Cycle Assess 22:111–124. https://doi.org/10.1007/s11367-015-0959-7
Boulanger AG, Chu AC, Maxx S, Waltz DL (2011) Vehicle electrification: status and issues. Proc IEEE 99(6):1116–1138. https://doi.org/10.1109/JPROC.2011.2112750
Brown S, Pyke D, Steenhof P (2010) Electric vehicles: the role and importance of standards in an emerging market. Energy Policy 38(7):3797–3806. https://doi.org/10.1016/j.enpol.2010.02.059
Chen T, Zhang X-P, Wang J, Li J, Wu C, Hu M, Bian H (2020) A review on electric vehicle charging infrastructure development in the UK. J Mod Power Syst Clean Energy 8(2):193–205. https://doi.org/10.35833/MPCE.2018.000374
Deilami S, Masoum AS, Moses PS, Masoum MAS (2011) Real-time coordination of plug-in electric vehicle charging in smart grids to minimize power losses and improve voltage profile. IEEE Trans Smart Grid 2(3):456–467. https://doi.org/10.1109/TSG.2011.2159816
Department of Energy, Office of Electricity (2022) The smart grid. https://www.smartgrid.gov/the_smart_grid/smart_grid.html . Accessed 14 Aug 2022.
Deng RL, Yang ZY, Chow MY, Chen JM (2015) A survey on demand response in smart grids: mathematical models and approaches. IEEE Trans Industr Inform 11(3):570–582. https://doi.org/10.1109/TII.2015.2414719
Denholm P, Eichman J, Markel T, Ma O (2015) Summary of market opportunities for electric vehicles and dispatchable load in electrolyzers (No. NREL/TP-6A20-64172). National Renewable Energy Lab, Golden, CO.
Donadee J, Ilie MD (2014) Stochastic optimization of grid to vehicle frequency regulation capacity bids. IEEE Trans Smart Grid 5(2):1061–1069. https://doi.org/10.1109/TSG.2013.2290971
Ehsani M, Falahi M, Lotfifard S (2012) Vehicle to grid services: potential and applications. Energies 5(10):4076–4090. https://doi.org/10.3390/en5104076
Erdinc O, Paterakis NG, Mendes TDP, Bakirtzis AG, Catalao JPS (2015) Smart household operation considering bi-directional EV and ESS utilization by real-time pricing-based DR. IEEE Trans Smart Grid 6(3):1281–1291. https://doi.org/10.1109/TSG.2014.2352650
Faulin J, Juan AA, Martorell S, Ramírez-Márquez J-E (2010) Simulation methods for reliability and availability of complex systems. Springer, London
Book MATH Google Scholar
Green RC II, Wang L, Alam M (2011) The impact of plug-in hybrid electric vehicles on distribution networks: a review and outlook. Renew Sust Energ Rev 15(1):544–553
He YF, Venkatesh B, Guan L (2012) Optimal scheduling for charging and discharging of electric vehicles. IEEE Trans Smart Grid 3(3):1095–1105. https://doi.org/10.1109/TSG.2011.2173507
Hernández-Moro J, Martínez-Duart JM (2012) CSP electricity cost evolution and grid parities based on the IEA roadmaps. Energy Policy 41:184–192. https://doi.org/10.1016/j.enpol.2011.10.032
Heymans C, Walker SB, Young SB, Fowler M (2014) Economic analysis of second use electric vehicle batteries for residential energy storage and load-levelling. Energy Policy 71:22–30. https://doi.org/10.1016/j.enpol.2014.04.016
Hu XS, Martinez CM, Yang YL (2017) Charging, power management, and battery degradation mitigation in plug-in hybrid electric vehicles: a unified cost-optimal approach. Mech Syst Signal Process 87:4–16. https://doi.org/10.1016/j.ymssp.2016.03.004
International Electrotechnical Commission (2012) Grid integration of large-capacity renewable energy sources and use of large-capacity electrical energy storage. White paper 3
Jian LN, Zheng YC, Xiao XP, Chan CC (2015) Optimal scheduling for vehicle-to-grid operation with stochastic connection of plug-in electric vehicles to smart grid. Appl Energy 146:150–161. https://doi.org/10.1016/j.apenergy.2015.02.030
Jin CR, Tang J, Ghosh P (2013) Optimizing electric vehicle charging with energy storage in the electricity market. IEEE Trans Smart Grid 4(1):311–320. https://doi.org/10.1109/TSG.2012.2218834
Kennel F, Görges D, Liu S (2013) Energy management for smart grids with electric vehicles based on hierarchical MPC. IEEE Trans Industr Inform 9(3):1528–1537. https://doi.org/10.1109/TII.2012.2228876
Kim B, Ren S, van der Schaar M, Lee J (2013) Bidirectional energy trading and residential load scheduling with electric vehicles in the smart grid. IEEE J Sel Areas Commun 31(7):1219–1234. https://doi.org/10.1109/JSAC.2013.130706
Kisacikoglu MC, Kesler M, Tolbert LM (2015) Single-phase on-board bidirectional PEV charger for V2G reactive power operation. IEEE Trans Smart Grid 6(2):767–775. https://doi.org/10.1109/TSG.2014.2360685
Kitchenham B (2004) Procedures for performing systematic reviews. Keele University Technical Report TR/SE-0401. Keele, UK. https://www.researchgate.net/profile/Barbara-Kitchenham/publication/228756057_Procedures_for_Performing_Systematic_Reviews . Accessed 27 Sep 2022.
Kitchenham B, Brereton OP, Budgen D, Turner M, Bailey J, Linkman S (2009) Systematic literature reviews in software engineering—a systematic literature review. Inf Softw Technol 51(1):7–15
Kong P, Karagiannidis GK (2016) Charging schemes for plug-in hybrid electric vehicles in smart grid: a survey. IEEE Access 4:6846–6875. https://doi.org/10.1109/ACCESS.2016.2614689
Lopez MA, de la Torre S, Martin S, Aguado JA (2015) Demand-side management in smart grid operation considering electric vehicles load shifting and vehicle-to-grid support. Int J Electr Power Energy Syst 64:689–698. https://doi.org/10.1016/j.ijepes.2014.07.065
Martinez CM, Hu XS, Cao DP, Velenis E, Gao B, Wellers M (2017) Energy management in plug-in hybrid electric vehicles: recent progress and a connected vehicles perspective. IEEE Trans Veh Technol 66(6):4534–4549. https://doi.org/10.1109/TVT.2016.2582721
Meintz A (2022) Electric Vehicle Grid Integration. National Renewable Energy Lab. https://www.nrel.gov/transportation/project-ev-grid-integration.html . Accessed 14 Aug 2022.
Meliani M, Barkany AE, Abbassi IE, Darcherif AM, Mahmoudi M (2021) Energy management in the smart grid: state-of-the-art and future trends. Int J Eng Bus Manag 13:18479790211032920. https://doi.org/10.1177/18479790211032920
Mou YT, Xing H, Lin ZY, Fu MY (2015) Decentralized optimal demand-side management for PHEV charging in a smart grid. IEEE Trans Smart Grid 6(2):726–736. https://doi.org/10.1109/TSG.2014.2363096
Mukherjee JC, Gupta A (2015) A review of charge scheduling of electric vehicles in smart grid. IEEE Syst J 9(4):1541–1553. https://doi.org/10.1109/JSYST.2014.2356559
Mullan J, Harries D, Bräunl T, Whitely S (2012) The technical, economic and commercial viability of the vehicle-to-grid concept. Energy Policy 48:394–406. https://doi.org/10.1016/j.enpol.2012.05.042
Mwasilu F, Justo JJ, Kim EK, Do TD, Jung JW (2014) Electric vehicles and smart grid interaction: a review on vehicle to grid and renewable energy sources integration. Renew Sust Energ Rev 34:501–516
Ota Y, Taniguchi H, Nakajima T, Liyanage KM, Baba J, Yokoyama A (2012) Autonomous distributed V2G (vehicle-to-grid) satisfying scheduled charging. IEEE Trans Smart Grid 3(1):559–564. https://doi.org/10.1109/TSG.2011.2167993
Pang C, Dutta P, Kezunovic M (2012) BEVs/PHEVs as dispersed energy storage for V2B uses in the smart grid. IEEE Trans Smart Grid 3(1):473–482. https://doi.org/10.1109/TSG.2011.2172228
Peterson SB, Whitacre JF, Apt J (2010) The economics of using plug-in hybrid electric vehicle battery packs for grid storage. J Power Sources 195(8):2377–2384. https://doi.org/10.1016/j.jpowsour.2009.09.070
Rames C, Wilson AM, Zimny-Schmitt D, Neri C, Sperling J, Romero-Lankao P (2021) A data-driven mobility–energy typology framework for New York State. Environ Plan B Urban Anal City Sci 48(8):2254–2271. https://doi.org/10.1177/2399808320974032
Rassaei F, Soh W, Chua K (2015) Demand response for residential electric vehicles with random usage patterns in smart grids. IEEE Trans Sustain Energy 6(4):1367–1376. https://doi.org/10.1109/TSTE.2015.2438037
Ren H, Zhang A, Wang F, Yan X, Li Y, Duić N, Shafie-Khah M, Catalão JP (2021) Optimal scheduling of an EV aggregator for demand response considering triple level benefits of three-parties. Int J Electr Power Energy Syst 125:106447. https://doi.org/10.1016/j.ijepes.2020.106447
Rigas ES, Ramchurn SD, Bassiliades N (2015) Managing electric vehicles in the smart grid using artificial intelligence: a survey. IEEE Trans Intell Transp Syst 16(4):1619–1635. https://doi.org/10.1109/TITS.2014.2376873
Rostami M, Kavousi-Fard A, Niknam T (2015) Expected cost minimization of smart grids with plug-in hybrid electric vehicles using optimal distribution feeder reconfiguration. IEEE Trans Industr Inform 11(2):388–397. https://doi.org/10.1109/TII.2015.2395957
San Diego Gas and Electric (2015) Electric vehicle grid integration. http://www.westernlampac.org/2015%20Spring%20Conference/Laura%20McDonald%20EVGI%20LAMPAC%20Presentation%20042715.pdf . Accessed 27 Sep 2022.
Sanghvi A, Markel T (2021) Cybersecurity for electric vehicle fast-charging infrastructure. In: IEEE Transportation Electrification Conference and Expo. 573–576. https://doi.org/10.1109/ITEC51675.2021.9490069
Sbordone D, Bertini I, Di Pietra B, Falvo MC, Genovese A, Martirano L (2015) EV fast charging stations and energy storage technologies: a real implementation in the smart micro grid paradigm. Electr Power Syst Res 120:96–108. https://doi.org/10.1016/j.epsr.2014.07.033
Shafie-khah M, Heydarian-Forushani E, Osorio GJ, Gil FAS, Aghaei J, Barani M, Catalao JPS (2016) Optimal behavior of electric vehicle parking lots as demand response aggregation agents. IEEE Trans Smart Grid 7(6):2654–2665. https://doi.org/10.1109/TSG.2015.2496796
Singh M, Kumar P, Kar I (2012) Implementation of vehicle to grid infrastructure using fuzzy logic controller. IEEE Trans Smart Grid 3(1):565–577. https://doi.org/10.1109/TSG.2011.2172697
Sojoudi S, Low SH (2011) Optimal charging of plug-in hybrid electric vehicles in smart grids. In: 2011 IEEE Power and Energy Society General Meeting. https://doi.org/10.1109/PES.2011.6039236
Spanos G, Angelis L (2016) The impact of information security events to the stock market: a systematic literature review. Comput Secur 58:216–229
Steckel T, Kendall A, Ambrose H (2021) Applying levelized cost of storage methodology to utility-scale second-life lithium-ion battery energy storage systems. Appl Energy 300:117309. https://doi.org/10.1016/j.apenergy.2021.117309
Su WC, Chow MY (2012a) Computational intelligence-based energy management for a large-scale PHEV/PEV enabled municipal parking deck. Appl Energy 96:171–182. https://doi.org/10.1016/j.apenergy.2011.11.088
Su WC, Chow MY (2012b) Performance evaluation of an EDA-based large-scale plug-in hybrid electric vehicle charging algorithm. IEEE Trans Smart Grid 3(1):308–315. https://doi.org/10.1109/TSG.2011.2151888
Su WC, Rahimi-Eichi H, Zeng WT, Chow MY (2012) A survey on the electrification of transportation in a smart grid environment. IEEE Trans Industr Inform 8(1):1–10. https://doi.org/10.1109/TII.2011.2172454
Su WC, Wang JH, Roh J (2014) Stochastic energy scheduling in microgrids with intermittent renewable energy resources. IEEE Trans Smart Grid 5(4):1876–1883. https://doi.org/10.1109/TSG.2013.2280645
Sultan V, Bitar H, Alzahrani A, Hilton B (2017) Electric vehicles charging infrastructure integration into the electric grid considering the net benefits to consumers. Energy 2017:21–26
Google Scholar
Tan Z, Yang P, Nehorai A (2014) An optimal and distributed demand response strategy with electric vehicles in the smart grid. IEEE Trans Smart Grid 5(2):861–869. https://doi.org/10.1109/TSG.2013.2291330
Tushar W, Saad W, Poor HV, Smith DB (2012) Economics of electric vehicle charging: a game theoretic approach. IEEE Trans Smart Grid 3(4):1767–1778. https://doi.org/10.1109/TSG.2012.2211901
Vachirasricirikul S, Ngamroo I (2014) Robust LFC in a smart grid with wind power penetration by coordinated V2G control and frequency controller. IEEE Trans Smart Grid. 5(1):371–380. https://doi.org/10.1109/TSG.2013.2264921
Van der Kam M, van Sark W (2015) Smart charging of electric vehicles with photovoltaic power and vehicle-to-grid technology in a microgrid: a case study. Appl Energy 152:20–30. https://doi.org/10.1016/j.apenergy.2015.04.092
Veldman E, Verzijlbergh RA (2015) Distribution grid impacts of smart electric vehicle charging from different perspectives. IEEE Trans Smart Grid 6(1):333–342. https://doi.org/10.1109/TSG.2014.2355494
Verzijlbergh RA, De Vries LJ, Lukszo Z (2014) Renewable energy sources and responsive demand. Do we need congestion management in the distribution grid? IEEE Trans Power Syst 29(5):2119–2128. https://doi.org/10.1109/TPWRS.2014.2300941
Wang GB, Xu Z, Wen FS, Wong KP (2013) Traffic-constrained multiobjective planning of electric-vehicle charging stations. IEEE Trans Power Deliv 28(4):2363–2372. https://doi.org/10.1109/TPWRD.2013.2269142
Wu CY, Mohsenian-Rad H, Huang JW (2012) Vehicle-to-aggregator interaction game. IEEE Trans Smart Grid 3(1):434–442. https://doi.org/10.1109/TSG.2011.2166414
Xing H, Fu M, Lin Z, Mou Y (2016) Decentralized optimal scheduling for charging and discharging of plug-in electric vehicles in smart grids. IEEE Trans Power Syst Adv Online Publ. https://doi.org/10.1109/TPWRS.2015.2507179
Yao L, Lim WH, Tsai TS (2017) A real-time charging scheme for demand response in electric vehicle parking stations. IEEE Trans Smart Grid 8(1):52–62. https://doi.org/10.1109/TSG.2016.2582749
Yu R, Zhong W, Xie S, Yuen C, Gjessing S, Zhang Y (2016) Balancing power demand through EV mobility in vehicle-to-grid mobile energy networks. IEEE Trans Industr Inform 12(1):79–90. https://doi.org/10.1109/TII.2015.2494884
Zhang MR, Chen J (2014) The energy management and optimized operation of electric vehicles based on microgrid. IEEE Trans Power Deliv 29(3):1427–1435. https://doi.org/10.1109/TPWRD.2014.2303492
Zhou H, Zhou Y, Hu J, Yang G, Denmark L, Xie D, Xue Y, Nordström L (2021) LSTM-based energy management for electric vehicle charging in commercial-building prosumers. J Mod Power Syst Clean Energy 9(5):1205–1216. https://doi.org/10.35833/MPCE.2020.000501
Download references
This research is based on students’ course project work at the College of Business & Economics of California State University, Los Angeles, where VS and AA are faculty in the Department of Information Systems, HC and JK are students in the undergraduate program. No additional funding was used for this project.
Author information
Authors and affiliations.
California State University, College of Business and Economics, Los Angeles, CA, 90032, USA
Vivian Sultan, Arun Aryal, Hao Chang & Jiri Kral
You can also search for this author in PubMed Google Scholar
Contributions
VS and AA initiated the research project, conducted the broad automated search, selected the digital sources, determined the search terms, administered and led the analysis, reviewed the findings, and proofread/edited the final paper. HC and JK completed the literature review. All authors read and approved the final manuscript.
Corresponding author
Correspondence to Vivian Sultan .
Ethics declarations
Ethical approval and consent to participate.
Not applicable.
Consent for publication
Competing interests.
The authors declare that they have no competing interests.
Additional information
Publisher's note.
Springer Nature remains neutral with regard to jurisdictional claims in published maps and institutional affiliations.
Rights and permissions
Open Access This article is licensed under a Creative Commons Attribution 4.0 International License, which permits use, sharing, adaptation, distribution and reproduction in any medium or format, as long as you give appropriate credit to the original author(s) and the source, provide a link to the Creative Commons licence, and indicate if changes were made. The images or other third party material in this article are included in the article's Creative Commons licence, unless indicated otherwise in a credit line to the material. If material is not included in the article's Creative Commons licence and your intended use is not permitted by statutory regulation or exceeds the permitted use, you will need to obtain permission directly from the copyright holder. To view a copy of this licence, visit http://creativecommons.org/licenses/by/4.0/ .
Reprints and permissions
About this article
Cite this article.
Sultan, V., Aryal, A., Chang, H. et al. Integration of EVs into the smart grid: a systematic literature review. Energy Inform 5 , 65 (2022). https://doi.org/10.1186/s42162-022-00251-2
Download citation
Received : 06 October 2022
Accepted : 08 December 2022
Published : 15 December 2022
DOI : https://doi.org/10.1186/s42162-022-00251-2
Share this article
Anyone you share the following link with will be able to read this content:
Sorry, a shareable link is not currently available for this article.
Provided by the Springer Nature SharedIt content-sharing initiative
- Electric vehicles
- Distributed storage
- Literature review
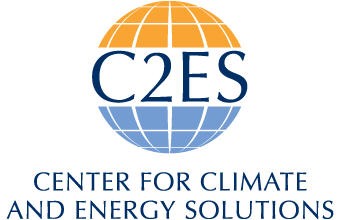
Library » Paper
Plug-in electric vehicles: literature review.
This paper reviews the current literature on plug-in electric vehicles (PEVs) with a focus on issues and solutions related to vehicle deployment and integration with the U.S. electrical grid. It is a companion to the Center’s “Plug-in Electric Vehicles Market: State of Play.” The subjects covered include vehicles, electricity, the passenger vehicle market, and public policy. This paper relies on the most recent research from government, private business, academia, and research institutions; peer-reviewed literature was used wherever possible. The paper’s purpose is to provide a foundation for overcoming some of the major hurdles to PEV deployment in the United States both currently and in the future.
Download Paper (pdf, 967 KB)
Advertisement
Consumer intention to accept electric two-wheelers in India: a valence theory approach to unveil the role of identity and utility
- Published: 22 September 2023
Cite this article
- Furqan A. Bhat 1 &
- Ashish Verma 1
465 Accesses
Explore all metrics
Two-wheelers in India account for more than 70% of the total motorised vehicles, with approximately half of the Indian households owning at least one two-wheeler as compared to 7.5% of the households owning a four-wheeler. An impetus towards electrification of mobility is crucial for India, given the enormous volume of oil imports and the subsequent negative impacts it has on the trade balance and the environment. However, the adoption rates of electric two-wheelers remain low, and hence, for fruitful penetration of electric two-wheelers in developing economies such as India, this study analyses the factors affecting the adoption behaviour of electric two-wheelers. This study uses data collected from 1375 potential electric two-wheeler buyers of Bengaluru, India, to study the influence of socio-demographic variables and certain latent factors such as environmental enthusiasm, technological enthusiasm, monetary benefits, environmental benefits, social image, lack of infrastructural readiness, perceived fee, and perceived risks on consumers’ intention to adopt electric two-wheelers. The results reveal environmental enthusiasm, technological enthusiasm, monetary benefits, environmental benefits, and social image to have a significant positive impact on electric two-wheeler adoption intention. Perceived fees and perceived risks are found to hinder the adoption of electric two-wheelers, while the lack of infrastructural facilities does not have a direct impact on the intention to adopt electric two-wheelers among potential buyers. Moreover, socio-demographic variables are also found to be significant determinants of electric vehicle adoption behaviour. This study provides some important implications for policy and decision-makers that can help in the widespread adoption of electric two-wheelers.
This is a preview of subscription content, log in via an institution to check access.
Access this article
Price includes VAT (Russian Federation)
Instant access to the full article PDF.
Rent this article via DeepDyve
Institutional subscriptions
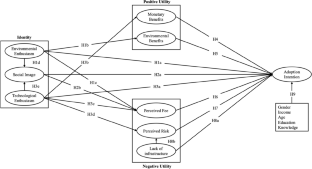
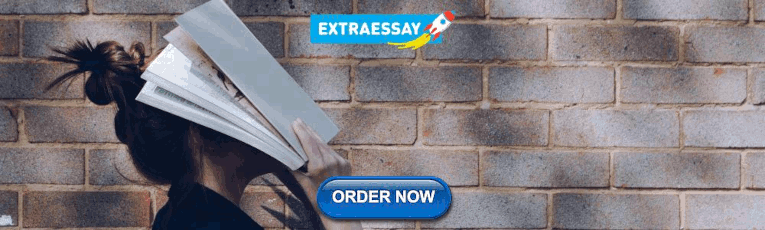
Similar content being viewed by others
Two-Wheeler Electric Vehicles Purchase Policy
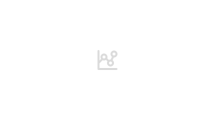
College students’ potential purchase intention of electric two-wheeled vehicles
Rong-Chang Jou, Chih-Hung Lai & Tzu-Ying Chen
Analysis of the Key Determinants of Electric Two-Wheeler Use in the Indian Context
Ab Hamid, M.R., Sami, W., Mohmad Sidek, M.H.: Discriminant validity assessment: use of Fornell & Larcker criterion versus HTMT criterion. In: Journal of Physics: Conference Series, vol. 890, p. 012163 (2017). https://doi.org/10.1088/1742-6596/890/1/012163
Adnan, N., Nordin, S.M., Rahman, I., Rasli, A.M.: A new era of sustainable transport: an experimental examination on forecasting adoption behavior of EVs among Malaysian consumer. Transp. Res. Part A Policy Pract. 103 , 279–295 (2017). https://doi.org/10.1016/j.tra.2017.06.010
Article Google Scholar
Ajzen, I.: The theory of planned behavior. Organ. Behav. Hum. Decis. Process. 50 , 179–211 (1991). https://doi.org/10.1016/0749-5978(91)90020-T
Alzahrani, K., Hall-Phillips, A., Zeng, A.Z.: Applying the theory of reasoned action to understanding consumers’ intention to adopt hybrid electric vehicles in Saudi Arabia. Transportation (amst). 46 , 199–215 (2019). https://doi.org/10.1007/s11116-017-9801-3
Asadi, S., Nilashi, M., Samad, S., Abdullah, R., Mahmoud, M., Alkinani, M.H., Yadegaridehkordi, E.: Factors impacting consumers’ intention toward adoption of electric vehicles in Malaysia. J. Clean. Prod. 282 , 124474 (2021). https://doi.org/10.1016/J.JCLEPRO.2020.124474
Austmann, L.M., Vigne, S.A.: Does environmental awareness fuel the electric vehicle market? A Twitter keyword analysis. Energy Econ. 101 , 105337 (2021). https://doi.org/10.1016/J.ENECO.2021.105337
Bang, H.K., Ellinger, A.E., Hadjimarcou, J., Traichal, P.A.: Consumer concern, knowledge, belief, and attitude toward renewable energy: an application of the reasoned action theory. Psychol. Mark. 17 , 449–468 (2000a). https://doi.org/10.1002/(SICI)1520-6793(200006)17:6%3c449::AID-MAR2%3e3.0.CO;2-8
Bansal, P., Kockelman, K.M.: Indian vehicle ownership: insights from literature review, expert interviews, and state-level model. In: Journal of the Transportation Research Forum, vol. 56 (2017)
Bansal, P., Kockelman, K.M., Schievelbein, W., Schauer-West, S.: Indian vehicle ownership and travel behavior: a case study of Bengaluru, Delhi and Kolkata. Res. Transp. Econ. 71 , 2–8 (2018). https://doi.org/10.1016/J.RETREC.2018.07.025
Bansal, P., Kumar, R.R., Raj, A., Dubey, S., Graham, D.J.: Willingness to pay and attitudinal preferences of Indian consumers for electric vehicles. Energy Econ. 100 , 105340 (2021). https://doi.org/10.1016/J.ENECO.2021.105340
van den Berg, P., Vinken, S., Geurs, K., Arentze, T.: Stated choice model of transport modes including solar bike. J. Transp. Land Use 11 , 901–919 (2018). https://doi.org/10.5198/JTLU.2018.1149
Bethlehem, J.: Selection bias in web surveys. Int. Stat. Rev. 78 , 161–188 (2010)
Bhat, F.A., Verma, A.: A bibliometric analysis and review of adoption behaviour of electric vehicles. Transp. Dev. Econ. 9 , 1–30 (2022). https://doi.org/10.1007/S40890-022-00175-2
Bhat, F.A., Verma, M., Verma, A.: Measuring and modelling electric vehicle adoption of Indian consumers. Transp. Dev. Econ. 8 , 6 (2022). https://doi.org/10.1007/s40890-021-00143-2
Bhatt, Y.: India’s Transport CO2 Emissions and Value Added Contribution—KAPSARC (2019), https://www.kapsarc.org/research/publications/indias-transport-co2-emissions-and-value-added-contribution/
Bhutani, C.: Govt ropes in IISc Bangalore to expand probe into electric scooter fire mishaps (2022)
Bilkey, W.J.: A psychological approach to consumer behavior analysis. J. Mark. 18 , 18–25 (1953). https://doi.org/10.1177/002224295301800103
Bjerkan, K.Y., Nørbech, T.E., Nordtømme, M.E.: Incentives for promoting Battery Electric Vehicle (BEV) adoption in Norway. Transp. Res. D Transp. Environ. 43 , 169–180 (2016). https://doi.org/10.1016/j.trd.2015.12.002
Carley, S., Krause, R.M., Lane, B.W., Graham, J.D.: Intent to purchase a plug-in electric vehicle: a survey of early impressions in large US cites. Transp. Res. D Transp. Environ. 18 , 39–45 (2013). https://doi.org/10.1016/j.trd.2012.09.007
Carley, S., Siddiki, S., Nicholson-Crotty, S.: Evolution of plug-in electric vehicle demand: assessing consumer perceptions and intent to purchase over time. Transp. Res. D Transp. Environ. 70 , 94–111 (2019). https://doi.org/10.1016/j.trd.2019.04.002
Chakraborty, R., Chakravarty, S.: Factors affecting acceptance of electric two-wheelers in India: a discrete choice survey. Transp. Policy (oxf) 132 , 27–41 (2023). https://doi.org/10.1016/J.TRANPOL.2022.12.015
Chandler, J., Sisso, I., Shapiro, D.: Participant carelessness and fraud: consequences for clinical research and potential solutions. J. Abnorm. Psychol. 129 , 49–55 (2020). https://doi.org/10.1037/ABN0000479
Chen, C.F., Eccarius, T., Su, P.C.: The role of environmental concern in forming intentions for switching to electric scooters. Transp. Res. Part A Policy Pract. 154 , 129–144 (2021). https://doi.org/10.1016/J.TRA.2021.10.010
Chen, C.F., Xu, X., Frey, S.: Who wants solar water heaters and alternative fuel vehicles? assessing social–psychological predictors of adoption intention and policy support in China. Energy Res. Soc. Sci. 15 , 1–11 (2016). https://doi.org/10.1016/J.ERSS.2016.02.006
Chhikara, R., Garg, R., Chhabra, S., Karnatak, U., Agrawal, G.: Factors affecting adoption of electric vehicles in India: an exploratory study. Transp. Res. D Transp. Environ. (2021). https://doi.org/10.1016/j.trd.2021.103084
Choudhary, G.: Another electric scooter caught on fire; no injuries reported | Mint, https://www.livemint.com/technology/tech-news/another-electric-scooter-caught-on-fire-no-injuries-reported-11670996679121.html (2022)
Chu, W., Im, M., Song, M.R., Park, J.: Psychological and behavioral factors affecting electric vehicle adoption and satisfaction: a comparative study of early adopters in China and Korea. Transp. Res. D Transp. Environ. 76 , 1–18 (2019). https://doi.org/10.1016/J.TRD.2019.09.009
Coffman, M., Bernstein, P., Wee, S.: Electric vehicles revisited: a review of factors that affect adoption. Transp. Rev. 37 , 79–93 (2017). https://doi.org/10.1080/01441647.2016.1217282
Dash, S., Vasudevan, V., Singh, S.: Disaggregate model for vehicle ownership behavior of Indian households. Transp. Res. Rec. (2013). https://doi.org/10.3141/2394-07
Davis, F.D.: Perceived usefulness, perceived ease of use, and user acceptance of information technology. MIS Q. 13 , 319–339 (1989). https://doi.org/10.2307/249008
Ding, C., Wang, Y., Tang, T., Mishra, S., Liu, C.: Joint analysis of the spatial impacts of built environment on car ownership and travel mode choice. Transp. Res. Part D 60 , 28–40 (2018)
Dogra, S.: In The Future, You Will Get Parking Only If You Drive An Electric Vehicle (2019), https://www.indiatimes.com/auto/current/karnataka-will-soon-reserve-20-percent-parking-for-electric-vehicles-378513.html
Duann, L.S., Chou, H.Y., Wang, C.C.: The factors affecting the demand for electric motorcycle. J. East. Asia Soc. Transp. Stud. 4 , 209–220 (2001)
Google Scholar
Dumortier, J., Siddiki, S., Carley, S., Cisney, J., Krause, R.M., Lane, B.W., Rupp, J.A., Graham, J.D.: Effects of providing total cost of ownership information on consumers’ intent to purchase a hybrid or plug-in electric vehicle. Transp. Res. Part A Policy Pract. 72 , 71–86 (2015). https://doi.org/10.1016/j.tra.2014.12.005
Eccarius, T., Lu, C.C.: Powered two-wheelers for sustainable mobility: a review of consumer adoption of electric motorcycles. Int. J. Sustain. Transp. 14 , 215–231 (2020). https://doi.org/10.1080/15568318.2018.1540735
Egbue, O., Long, S.: Barriers to widespread adoption of electric vehicles: an analysis of consumer attitudes and perceptions. Energy Policy 48 , 717–729 (2012). https://doi.org/10.1016/j.enpol.2012.06.009
Eppstein, M.J., Grover, D.K., Marshall, J.S., Rizzo, D.M.: An agent-based model to study market penetration of plug-in hybrid electric vehicles. Energy Policy 39 , 3789–3802 (2011). https://doi.org/10.1016/j.enpol.2011.04.007
Eswar, M., Roy, A.K.: Urbanisation in Karnataka: trend and spatial pattern. J. Reg. Dev. Plan. 5 , 5–20 (2018). https://doi.org/10.1080/17441730902790024
Fishbein, M., Ajzen, I.: Beliefs, Attitude, Intention and Behavior: An Introduction to Theory and Research. Addison-Wesley, Reading (1975)
Fujii, S.: Environmental concern, attitude toward frugality, and ease of behavior as determinants of pro-environmental behavior intentions. J. Environ. Psychol. 26 , 262–268 (2006). https://doi.org/10.1016/j.jenvp.2006.09.003
Garbarino, E., Strahilevitz, M.: Gender differences in the perceived risk of buying online and the effects of receiving a site recommendation. J. Bus. Res. 57 , 768–775 (2004). https://doi.org/10.1016/S0148-2963(02)00363-6
Garretson, J.A., Clow, K.E.: The influence of coupon face value on service quality expectations, risk perceptions and purchase intentions in the dental industry. J. Serv. Mark. 13 , 59–72 (1999). https://doi.org/10.1108/08876049910256122
Goel, R., Mohan, D.: Investigating the association between population density and travel patterns in Indian cities—an analysis of 2011 census data. Cities 100 , 102656 (2020). https://doi.org/10.1016/J.CITIES.2020.102656
Goel, S., Sharma, R., Rathore, A.K.: A review on barrier and challenges of electric vehicle in India and vehicle to grid optimisation. Transp. Eng. 4 , 100057 (2021). https://doi.org/10.1016/J.TRENG.2021.100057
GoI: FAME-I: Scheme for faster adoption and manufacturing of (hybrid &) electric vehicles in India. New Delhi (2015)
Gopisetty, V., Srinivasan, K.K.: Joint models for analysis of household trip frequency and vehicle ownership in Chennai city. Int. J. Adv. Eng. Sci. Appl. Math. 5 , 129–144 (2013). https://doi.org/10.1007/s12572-013-0091-5
Guahroya, A., Majumdarb, B.B., Sahc, P.K., Majid, A.: Identification and evaluation of key determinants of two-wheeler ownership model in the context of developing countries: a case study of Pune city, India. J. East. Asia Soc. Transp. Stud. 13 , 120–131 (2019)
Guerra, E.: Electric vehicles, air pollution, and the motorcycle city: a stated preference survey of consumers’ willingness to adopt electric motorcycles in Solo, Indonesia. Transp. Res. D Transp. Environ. 68 , 52–64 (2019). https://doi.org/10.1016/J.TRD.2017.07.027
Bang, H.-K., Ellinger, A.E., Hadjimarcou, J., Traichal, P.A.: Consumer concern, knowledge, belief, and attitude toward renewable energy: an application of the reasoned action theory—Bang—2000—Psychology & Marketing—Wiley Online Library. Psychol. Mark. 17 , 449–468 (2000b)
Hair, J.F., Sarstedt, M., Hopkins, L., Kuppelwieser, V.G.: Partial least squares structural equation modeling (PLS-SEM): an emerging tool in business research. Eur. Bus. Rev. 26 , 106–121 (2014)
Hair, J.H., Balck, W.C., Babin, B.J., Anderson, R.E.: Multivariate data analysis: a global perspective. Prentice Hall, Upper Saddle River (2009)
Han, L., Wang, S., Zhao, D., Li, J.: The intention to adopt electric vehicles: driven by functional and non-functional values. Transp. Res. Part A Policy Pract. 103 , 185–197 (2017). https://doi.org/10.1016/j.tra.2017.05.033
He, X., Zhan, W., Hu, Y.: Consumer purchase intention of electric vehicles in China: the roles of perception and personality. J. Clean Prod. 204 , 1060–1069 (2018). https://doi.org/10.1016/j.jclepro.2018.08.260
Hidrue, M.K., Parsons, G.R., Kempton, W., Gardner, M.P.: Willingness to pay for electric vehicles and their attributes. Resour. Energy Econ. 33 , 686–705 (2011). https://doi.org/10.1016/j.reseneeco.2011.02.002
Hung, S.Y., Ku, C.Y., Chang, C.M.: Critical factors of WAP services adoption: an empirical study. Electron. Commerce Res. Appl. 2 , 42–60 (2003). https://doi.org/10.1016/S1567-4223(03)00008-5
IEA: World Energy Outlook 2009, IEA, Paris (2009)
IEA: Tracking Transport 2020, IEA, Paris (2020)
International Institute for Population Sciences, ICF: National Family Health Survey (NFHS-5), 2019–21. Mumbai (2021)
Jain, N.K., Bhaskar, K., Jain, S.: What drives adoption intention of electric vehicles in India? an integrated UTAUT model with environmental concerns, perceived risk and government support. Res. Transp. Bus. Manag. (2022a). https://doi.org/10.1016/j.rtbm.2021.100730
Jain, N.K., Bhaskar, K., Jain, S.: What drives adoption intention of electric vehicles in India? An integrated UTAUT model with environmental concerns, perceived risk and government support. Res. Transp. Bus. Manag. 42 , 100730 (2022b). https://doi.org/10.1016/J.RTBM.2021.100730
Jaiswal, D., Deshmukh, A.K., Thaichon, P.: Who will adopt electric vehicles? Segmenting and exemplifying potential buyer heterogeneity and forthcoming research. J. Retail. Consum. Serv. 67 , 102969 (2022). https://doi.org/10.1016/J.JRETCONSER.2022.102969
Jansson, J., Marell, A., Nordlund, A.: Green consumer behavior: determinants of curtailment and eco-innovation adoption. J. Consum. Mark. 27 , 358–370 (2010). https://doi.org/10.1108/07363761011052396
Cao, X., Næss, P., Wolday, F.: Examining the effects of the built environment on auto ownership in two Norwegian urban regions. Transp. Res. D Transp. Environ. 67 , 464–474 (2019). https://doi.org/10.1016/J.TRD.2018.12.020
Jensen, A.F., Cherchi, E., Mabit, S.L.: On the stability of preferences and attitudes before and after experiencing an electric vehicle. Transp. Res. D Transp. Environ. 25 , 24–32 (2013). https://doi.org/10.1016/j.trd.2013.07.006
Jindel, J., Chandra, A., Allirani, H., Verma, A.: Studying two-wheeler usage in the context of sustainable and resilient urban mobility policies in India. Transp. Res. Rec. 2676 , 424–436 (2022). https://doi.org/10.1177/03611981221074644
Junquera, B., Moreno, B., Álvarez, R.: Analyzing consumer attitudes towards electric vehicle purchasing intentions in Spain: technological limitations and vehicle confidence. Technol. Forecast Soc. Change 109 , 6–14 (2016). https://doi.org/10.1016/j.techfore.2016.05.006
Ken Research: Malaysia Electric Two-Wheeler Market Future Outlook to 2026: Driven by increase in awareness about alternative fuel, advancements in battery technology, and growing demand of two-wheelers in B2B Services (2022)
Kidiyoor, S.: Bengaluru’s number of vehicles doubles in a decade, but BMTC fleet size remains stagnant, https://www.thehindu.com/news/cities/bangalore/bengalurus-number-of-vehicles-doubles-in-a-decade-but-bmtc-fleet-size-remains-stagnant/article65442021.ece (2022)
Kim, J., Rasouli, S., Timmermans, H.: Expanding scope of hybrid choice models allowing for mixture of social influences and latent attitudes: application to intended purchase of electric cars. Transp. Res. Part A Policy Pract. 69 , 71–85 (2014). https://doi.org/10.1016/j.tra.2014.08.016
Kresnanto, N.C.: Model of relationship between car ownership growth and economic growth in Java. In: IOP Conference Series: Materials Science and Engineering, vol. 650, p. 012047 (2019). https://doi.org/10.1088/1757-899X/650/1/012047
Kwon, Y., Son, S., Jang, K.: User satisfaction with battery electric vehicles in South Korea. Transp. Res. D Transp. Environ. (2020). https://doi.org/10.1016/J.TRD.2020.102306
Lane, B., Potter, S.: The adoption of cleaner vehicles in the UK: exploring the consumer attitude-action gap. J. Clean Prod. 15 , 1085–1092 (2007). https://doi.org/10.1016/j.jclepro.2006.05.026
Le, H., Posada, F., Yang, Z.: Electric two-wheeler market growth in Vietnam: An overview. International Council on Clean Trasnportation (2022)
Le, H., Yang, Z.: Market analysis of two-and three-wheeler vehicles in key ASEAN member states. International Council on Clean Transportation (2022)
Levine, M.D., Koomey, J.G., McMahon, J.E., Sanstad, A.H., Hirst, E.: Energy efficiency policy and market failures. Annu. Rev. Energy Environ. 20 , 535–555 (1995). https://doi.org/10.1146/annurev.eg.20.110195.002535
Li, L., Wang, Z., Xie, X.: From government to market? A discrete choice analysis of policy instruments for electric vehicle adoption. Transp. Res. Part A Policy Pract. 160 , 143–159 (2022). https://doi.org/10.1016/J.TRA.2022.04.004
Liao, F., Molin, E., van Wee, B.: Consumer preferences for electric vehicles: a literature review. Transp. Rev. 37 , 252–275 (2017). https://doi.org/10.1080/01441647.2016.1230794
Liu, F., Zhao, X., Chau, P.Y.K., Tang, Q.: Roles of perceived value and individual differences in the acceptance of mobile coupon applications. Internet Res. 25 , 471–495 (2015). https://doi.org/10.1108/INTR-02-2014-0053
Maat, K., Timmermans, H.J.P.: Influence of the residential and work environment on car use in dual-earner households. Transp. Res. Part A Policy Pract. 43 , 654–664 (2009). https://doi.org/10.1016/J.TRA.2009.06.003
MacCallum, R.C., Browne, M.W., Sugawara, H.M.: Power analysis and determination of sample size for covariance structure modeling. Psychol. Methods 1 , 130–149 (1996)
Melliger, M.A., van Vliet, O.P.R., Liimatainen, H.: Anxiety vs reality—sufficiency of battery electric vehicle range in Switzerland and Finland. Transp. Res. D Transp. Environ. 65 , 101–115 (2018). https://doi.org/10.1016/j.trd.2018.08.011
Ministry of Environment Forest and Climate Change Government of India: India’s Long-Term Low-Carbon Development Strategy (2022)
MoRTH: Ministry of Road Transport and Highways Annual report 2020–2021. (2020)
Murugan, M., Marisamynathan, S.: Estimation of two-wheeler users’ mode shift behavior and policy analysis to encourage electric-bike adoption in India. Case Stud. Transp. Policy. (2022a). https://doi.org/10.1016/J.CSTP.2022.06.006
Murugan, M., Marisamynathan, S.: Mode shift behaviour and user willingness to adopt the electric two-wheeler: a study based on Indian road user preferences. Int. J. Transp. Sci. Technol. (2022b). https://doi.org/10.1016/J.IJTST.2022.03.008
Okada, T., Tamaki, T., Managi, S.: Effect of environmental awareness on purchase intention and satisfaction pertaining to electric vehicles in Japan. Transp. Res. D Transp. Environ. 67 , 503–513 (2019). https://doi.org/10.1016/J.TRD.2019.01.012
Ozaki, R., Sevastyanova, K.: Going hybrid: an analysis of consumer purchase motivations. Energy Policy 39 , 2217–2227 (2011)
Ozturk, A.B., Bilgihan, A., Salehi-Esfahani, S., Hua, N.: Understanding the mobile payment technology acceptance based on valence theory: a case of restaurant transactions. Int. J. Contemp. Hosp. Manag. 29 , 2027–2049 (2017). https://doi.org/10.1108/IJCHM-04-2016-0192/FULL/PDF
Pagiaslis, A., Krontalis, A.K.: Green consumption behavior antecedents: environmental concern, knowledge, and beliefs. Psychol. Mark. 31 , 335–348 (2014). https://doi.org/10.1002/mar.20698
Patil, M., Majumdar, B.B.: An investigation on the key determinants influencing electric two-wheeler usage in urban Indian context. Res. Transp. Bus. Manag. 43 , 100693 (2022). https://doi.org/10.1016/J.RTBM.2021.100693
Patil, M., Majumdar, B.B., Sahu, P.K., Truong, L.T.: Evaluation of prospective users’ choice decision toward electric two-wheelers using a stated preference survey: an indian perspective. Sustainability 13 , 3035 (2021). https://doi.org/10.3390/SU13063035
Pearre, N.S., Kempton, W., Guensler, R.L., Elango, V.V.: Electric vehicles: How much range is required for a day’s driving? Transp. Res. Part C Emerg. Technol. 19 , 1171–1184 (2011). https://doi.org/10.1016/j.trc.2010.12.010
Peter, J.P., Tarpey, L.X., Sr.: A comparative analysis of three consumer decision strategies. J. Consum. Res. 2 , 29–37 (1975). https://doi.org/10.1086/208613
Preacher, K.J., Coffman, D.L.: Computing power and minimum sample size for RMSEA [Computer software] (2006), http://www.quantpsy.org/rmsea/rmsea.htm
Rezvani, Z., Jansson, J., Bodin, J.: Advances in consumer electric vehicle adoption research: a review and research agenda. Transp. Res. D Transp. Environ. 34 , 122–136 (2015). https://doi.org/10.1016/j.trd.2014.10.010
Roychowdhury, A., Dubey, G.: The urban commute: And how it contributes to pollution and energy consumption. (2018)
Rudra, N.: Are Recent Fire Incidents Impacting Growth and Sales of EVs? Find Out What OEMs and Industry Associations Say (2022), https://circuitdigest.com/article/electric-vehicle-growth-and-sales-in-india
Sahoo, D., Harichandan, S., Kar, S.K., Sreejesh, S.: An empirical study on consumer motives and attitude towards adoption of electric vehicles in India: Policy implications for stakeholders. Energy Policy (2022). https://doi.org/10.1016/j.enpol.2022.112941
Sajjad, A., Asmi, F., Chu, J., Anwar, M.A.: Environmental concerns and switching toward electric vehicles: geographic and institutional perspectives. Environ. Sci. Pollut. Res. 27 , 39774–39785 (2020). https://doi.org/10.1007/s11356-020-08311-4
Sau Mei, N., Weng Wai, C., Ahamad, R.: Environmental awareness and practice in reducing climate change among Malaysian community. Procedia Soc. Behav. Sci. 222 , 668–675 (2016). https://doi.org/10.1016/j.sbspro.2016.05.223
Schleich, J.: Barriers to energy efficiency: a comparison across the German commercial and services sector. Ecol. Econ. 68 , 2150–2159 (2009)
Schuitema, G., Anable, J., Skippon, S., Kinnear, N.: The role of instrumental, hedonic and symbolic attributes in the intention to adopt electric vehicles. Transp. Res. Part A Policy Pract. 48 , 39–49 (2013). https://doi.org/10.1016/j.tra.2012.10.004
Schwartz, S.H.: Normative influences on altruism. Adv. Exp. Soc. Psychol. 10 , 221–279 (1977). https://doi.org/10.1016/S0065-2601(08)60358-5
Schwartz, S.H.: An overview of the Schwartz theory of basic values. Online Read Psychol Cult 2 , 11 (2012). https://doi.org/10.9707/2307-0919.1116
Scorrano, M., Rotaris, L.: The role of environmental awareness and knowledge in the choice of a seated electric scooter. Transp. Res. Part A Policy Pract. 160 , 333–347 (2022). https://doi.org/10.1016/J.TRA.2022.04.007
Scroll.in: Making two-wheelers less polluting to combat air pollution in India (2017f), https://scroll.in/b/105
Sen, S.: With 26 Lakh 2-wheelers, City Has 1,300 Bikes/km, https://timesofindia.indiatimes.com/city/mumbai/with-26-lakh-2-wheelers-city-has-1300-bikes/km/articleshow/94848415.cms , (2022)
Sharma, A.: Scooter Story: Asia’s Two Wheel EV Transition, https://icg.citi.com/icghome/what-we-think/global-insights/insights/scooter-story-asia-s-two-wheel-ev-transition
Sheeran, P.: Intention—behavior relations: a conceptual and empirical review. Eur Rev Soc Psychol 12 , 1–36 (2011). https://doi.org/10.1080/14792772143000003
Sheth, J.N., Newman, B.I., Gross, B.L.: Why we buy what we buy: a theory of consumption values. J. Bus. Res. 22 , 159–170 (1991). https://doi.org/10.1016/0148-2963(91)90050-8
Siegrist, M.: The influence of trust and perceptions of risks and benefits on the acceptance of gene technology. Risk Anal. 20 , 195–204 (2000). https://doi.org/10.1111/0272-4332.202020
Sierzchula, W.: Factors influencing fleet manager adoption of electric vehicles. Transp. Res. D Transp. Environ. 31 , 126–134 (2014). https://doi.org/10.1016/j.trd.2014.05.022
Sierzchula, W., Bakker, S., Maat, K., Van Wee, B.: The influence of financial incentives and other socio-economic factors on electric vehicle adoption. Energy Policy 68 , 183–194 (2014). https://doi.org/10.1016/j.enpol.2014.01.043
Singh, P.: E-two wheelers can cut carbon emissions in a big way, https://www.dailypioneer.com/2022/columnists/e-two-wheelers-can-cut-carbon-emissions-in-a-big-way.html , (2022)
Singh, S., Sagar, R.: A critical look at online survey or questionnaire-based research studies during COVID-19. Asian J. Psychiatr. 65 , 102850 (2021). https://doi.org/10.1016/J.AJP.2021.102850
Singh, V., Singh, V., Vaibhav, S.: A review and simple meta-analysis of factors influencing adoption of electric vehicles. Transp. Res. D Transp. Environ. (2020a). https://doi.org/10.1016/j.trd.2020.102436
Singh, V., Singh, V., Vaibhav, S.: A review and simple meta-analysis of factors influencing adoption of electric vehicles. Transp .res. D Transp. Environ. (2020b). https://doi.org/10.1016/J.TRD.2020.102436
Sinnappan, P., Rahman, A.A.: Antecedents of green purchasing behavior among Malaysian consumers. Int. Bus. Manag. 5 , 129–139 (2011). https://doi.org/10.3923/ibm.2011.129.139
Sivapriyan, E.: E-bike catches fire in Tamil Nadu’s Hosur; 29-year-old escapes unhurt, (2022)
Skippon, S.M., Kinnear, N., Lloyd, L., Stannard, J.: How experience of use influences mass-market drivers’ willingness to consider a battery electric vehicle: a randomised controlled trial. Transp. Res. Part A Policy Pract. 92 , 26–42 (2016). https://doi.org/10.1016/j.tra.2016.06.034
Srinivasan, K.K., Bhargavi, A.P.: Longer-term changes in mode choice decisions in Chennai: a comparison between cross-sectional and dynamic models. Transportation 34 , 355–374 (2007). https://doi.org/10.1007/s11116-007-9116-x
Sundaram, R.: Fire accidents burn down sales of electric vehicles in Tamil Nadu | Chennai News - Times of India (2022), https://timesofindia.indiatimes.com/city/chennai/fire-accidents-burn-down-sales-of-electric-vehicles-in-tamil-nadu/articleshow/91755912.cms
Sweeney, J.C., Soutar, G.N.: Consumer perceived value: the development of a multiple item scale. J. Retail. 77 , 203–220 (2001). https://doi.org/10.1016/S0022-4359(01)00041-0
The Economic Times: Over 21 crore two-wheelers, 7 crore four-wheelers and above category of vehicles registered in India (2022), https://economictimes.indiatimes.com/industry/auto/two-wheelers-three-wheelers/over-21-crore-two-wheelers-7-crore-four-wheelers-and-above-category-of-vehicles-registered-in-india/articleshow/93351004.cms?from=mdr
TIFAC, NITI Aayog: Forecasting penetration of electric two-wheelers in India—A bottom-up analysis (2022)
Turrentine, T.S., Kurani, K.S.: Car buyers and fuel economy? Energy Policy 35 , 1213–1223 (2007). https://doi.org/10.1016/j.enpol.2006.03.005
United Nations Climate Change: Key aspects of the Paris Agreement (2016), https://unfccc.int/most-requested/key-aspects-of-the-paris-agreement
Ursachi, G., Horodnic, I.A., Zait, A.: How reliable are measurement scales? External factors with indirect influence on reliability estimators. Procedia Econ. Finance 20 , 679–686 (2015). https://doi.org/10.1016/S2212-5671(15)00123-9
U.S. EIA: International Energy Outlook 2016. (2017)
Vajjarapu, H., Verma, A., Allirani, H.: Evaluating the climate change mitigation potential of sustainable urban transport measures in India. J. Urban Plan Dev. 149 , 04022047 (2023). https://doi.org/10.1061/(ASCE)UP.1943-5444.0000890
Venkadavarahan, M., Marisamynathan, S.: Estimation of rider’s shifting intention for electric bike adoption: An integrated choice and latent variable approach. Transp. Lett. (2022). https://doi.org/10.1080/19427867.2021.2000815
Venkatesh, V., Morris, M.G., Davis, G.B., Davis, F.D.: User acceptance of information technology: toward a unified view. MIS Q. 27 , 425–478 (2003)
Verma, M., Manoj, M., Verma, A.: Analysis of the influences of attitudinal factors on car ownership decisions among urban young adults in a developing country like India. Transp. Res. Part F Traffic Psychol. Behav. 42 , 90–103 (2016). https://doi.org/10.1016/J.TRF.2016.06.024
Verma, V.K., Chandra, B., Kumar, S.: Values and ascribed responsibility to predict consumers’ attitude and concern towards green hotel visit intention. J. Bus. Res. 96 , 206–216 (2019). https://doi.org/10.1016/J.JBUSRES.2018.11.021
Wahab, L., Jiang, H.: Factors influencing the adoption of electric vehicle: the case of electric motorcycle in northern Ghana. Int. J. Traffic Transp. Eng. 9 , 22–37 (2019). https://doi.org/10.7708/IJTTE.2019.9(1).03
Wang, N., Tang, L., Pan, H.: Analysis of public acceptance of electric vehicles: an empirical study in Shanghai. Technol. Forecast Soc. Change 126 , 284–291 (2018). https://doi.org/10.1016/j.techfore.2017.09.011
Wang, S., Fan, J., Zhao, D., Yang, S., Fu, Y.: Predicting consumers’ intention to adopt hybrid electric vehicles: using an extended version of the theory of planned behavior model. Transportation (amst). 43 , 123–143 (2016). https://doi.org/10.1007/s11116-014-9567-9
Wang, S., Li, J., Zhao, D.: The impact of policy measures on consumer intention to adopt electric vehicles: evidence from China. Transp. Res. Part A Policy Pract. 105 , 14–26 (2017). https://doi.org/10.1016/J.TRA.2017.08.013
Wedagama, D.M.P.: A multinomial logit model for estimating the influence of household characteristics on motorcycle ownership: a case study in Denpasar city Bali. J. Civ. Eng. 29 , 2–9 (2009)
White, L.V., Sintov, N.D.: You are what you drive: Environmentalist and social innovator symbolism drives electric vehicle adoption intentions. Transp. Res. Part A Policy Pract. 99 , 94–113 (2017). https://doi.org/10.1016/J.TRA.2017.03.008
World Population Review: Bangalore Population, https://worldpopulationreview.com/world-cities/bangalore-population
Yamamoto, T.: Comparative analysis of household car, motorcycle and bicycle ownership between Osaka metropolitan area, Japan and Kuala Lumpur, Malaysia. Transportation (amst.) 36 , 351–366 (2009)
Yeung, S.P.M.: Teaching approaches in geography and students’ environmental attitudes. Environmentalist 24 , 101–117 (2004). https://doi.org/10.1007/s10669-004-4801-1
Zegras, C.: The built environment and motor vehicle ownership and use: evidence from Santiago de Chile. Urban Stud. 47 , 1793–1817 (2010). https://doi.org/10.1177/0042098009356125
Zegras, P.C., Srinivasan, S.: Household income, travel behavior, location, and accessibility: sketches from two different developing contexts. Transp. Res. Rec. (2007). https://doi.org/10.3141/2038-17
Zhu, L., Song, Q., Sheng, N., Zhou, X.: Exploring the determinants of consumers’ WTB and WTP for electric motorcycles using CVM method in Macau. Energy Policy 127 , 64–72 (2019). https://doi.org/10.1016/J.ENPOL.2018.12.004
Download references
Acknowledgements
The authors would like to thank Ruth Babbington, Amy Davis, Domas Zemaitis, Sandhya Nagaraju, Chris Harrison, Sathsara Abeysinghe, Mo Abrahams and Thomas Gofrey-Brown from Energy Systems Catapult for their inputs throughout the project. The authors would also like to thank Eclat engineering private limited for their assistance in data collection. The authors acknowledge the important contribution made by Poornashree, Anusha, Atish, Bhumika, Deeksha, Ann and Vaibhav in data collection and processing. The authors would also like to thank the three anonymous reviewers for devoting the time and effort necessary to review the manuscript. We sincerely appreciate all valuable comments and suggestions, which helped us to significantly improve the quality of the manuscript.
The authors thank UK Research and Innovation (UKRI) SP/ESCL-21-0001 for the financial support.
Author information
Authors and affiliations.
Department of Civil Engineering, Indian Institute of Science, Bangalore, 560012, India
Furqan A. Bhat & Ashish Verma
You can also search for this author in PubMed Google Scholar
Contributions
The authors confirm contribution to the paper as follows: study conception and design: Bhat and Verma; Data collection: Bhat and Verma; Analysis: Bhat; Interpretation of results: Bhat and Verma; Draft manuscript preparation and revision: Bhat and Verma. All authors reviewed the results and approved the final version of the manuscript.
Corresponding author
Correspondence to Ashish Verma .
Ethics declarations
Competing interest.
The authors declare that they have no known competing financial interests or personal relationships that could have appeared to influence the work reported in this paper.
Additional information
Publisher's note.
Springer Nature remains neutral with regard to jurisdictional claims in published maps and institutional affiliations.
Rights and permissions
Springer Nature or its licensor (e.g. a society or other partner) holds exclusive rights to this article under a publishing agreement with the author(s) or other rightsholder(s); author self-archiving of the accepted manuscript version of this article is solely governed by the terms of such publishing agreement and applicable law.
Reprints and permissions
About this article
Bhat, F.A., Verma, A. Consumer intention to accept electric two-wheelers in India: a valence theory approach to unveil the role of identity and utility. Transportation (2023). https://doi.org/10.1007/s11116-023-10430-z
Download citation
Accepted : 08 September 2023
Published : 22 September 2023
DOI : https://doi.org/10.1007/s11116-023-10430-z
Share this article
Anyone you share the following link with will be able to read this content:
Sorry, a shareable link is not currently available for this article.
Provided by the Springer Nature SharedIt content-sharing initiative
- Electric vehicles (EVs)
- Electric two-wheelers
- Confirmatory factor analysis (CFA)
- Structural equation modelling (SEM)
- Adoption behaviour
- Find a journal
- Publish with us
- Track your research
- Skip to main content
- Keyboard shortcuts for audio player

- LISTEN & FOLLOW
- Apple Podcasts
- Google Podcasts
- Amazon Music
Your support helps make our show possible and unlocks access to our sponsor-free feed.
You asked, we answered: Your questions about electric vehicles

Camila Domonoske

If you're thinking about getting an electric vehicle, you're not alone.
People in the U.S. buy more than a million new cars every month, and as of March, less than 10% of those are electric vehicles. But more than half of car shoppers are at least considering battery-powered cars and SUVs, according to multiple studies .
And shoppers have lots of questions. In January, The Sunday Story, an NPR podcast, asked listeners for their EV questions. More than 60 listeners sent in queries, and The Sunday Story and Life Kit teamed up to answer them. The listener questions have been edited for length and clarity.
Are EVs truly better for the planet, even with mining for batteries and fossil-fuel -based electricity to charge them? (This was the No. 1 question asked by our listeners.)
The answer is yes . Many researchers have confirmed it , and online tools let you compare the impacts for yourself. One of the most recent analyses comes from Corey Cantor with the energy research company BloombergNEF, who headlined his report last month: "No Doubt About It: EVs Really Are Cleaner Than Gas Cars."
"Big picture, moving away from spewing more CO2 into the atmosphere is a good thing for the climate," he says. And the environmental benefits of EVs are getting bigger over time as grids get cleaner.
The Electric Car Race! Vroom, Vroom!

- Amazon Alexa
Is it better from an environmental standpoint to buy an electric vehicle now, or keep driving the gas car you have until you need a new car? –Ali Mercural, Portland, Ore.
For the climate, there's a strong case for switching now.
Yes, creating that new EV — getting the materials to build it from scratch — is resource-intensive. But the climate impact of a gas-powered car increases every single day you drive it.
To be precise, more than 85% of a gas-powered vehicle's lifetime emissions come from using the car, not from building the car. That's according to researchers at Argonne National Laboratory. And that means the new EV, despite its manufacturing costs, will be cleaner over time.
Jessika Trancik , a professor at the Institute for Data, Systems and Society at Massachusetts Institute of Technology, suggests taking the long view on decisions like these. Think not just about emissions right now but over the entire time you'll own a vehicle.
"Generally speaking, switching to that electric vehicle is going to provide a benefit over the lifetime of the car," she says.
I'm not proud, but I've run out of gas twice in my life. Luckily, I had friends nearby to bring me a gallon of gas. What would happen if I ran out of charge in an EV? Would a tow truck come to charge me up? How long would that take? And how embarrassing would that be? –Robin Rzechula, Chicago
We can't promise it won't be embarrassing, but a tow truck could tow you to a charger. In some cities, AAA will bring a mobile charger to you.
Overall, charging is a different experience than fueling up. With a combustion engine, you have to regularly make a stop at a gas station to fill up. With an EV, for daily driving, most people charge at home overnight – which drivers frequently cite as a major perk of EV ownership. (This does require the ability to charge at home).
For road trips, on the other hand, many parts of the country still have limited availability of fast chargers, which are high-speed chargers designed for use in the middle of a trip. Charger speeds and reliability at public charging stations vary, and charging takes much longer than filling up at a gas station.
So charging takes less work day-to-day, but more planning on long trips. Map out chargers on your route so you won't find yourself calling AAA.
Does leasing an electric car come with the same perks (like tax rebates) as buying an electric car? –Hallie Andrews, Washington, D.C.
The same or better.
There's a federal $7,500 tax credit for purchasing an EV, now available as an up-front credit toward the cost of the car. But the list of vehicles that qualify is short because of requirements meant to support U.S. jobs and supply chains. Buyers also have to be under an income cap.
Leased electric vehicles all qualify for a $7,500 credit – no matter where they're built, with no income cap. Check your lease paperwork to confirm that the credit is being fully passed along to you.
Efforts underway to make cities more EV-friendly
Wouldn't it be better to design cities around mass transit and use mass transit than get everyone to convert to electric vehicles? – Thomas Guffey, Los Angeles
Yes, designing cities to encourage mass transit – and to make them more walkable and bikeable – has a lower carbon footprint than relying on electric vehicles, in addition to other benefits . Electric bikes also have a fraction of the environmental footprint of EVs.
Switching to EVs is an important part of fighting climate change, but far from the only change that needs to happen.
The digital story was edited by Malaka Gharib. The visual editor is Beck Harlan. We'd love to hear from you. Leave us a voicemail at 202-216-9823, or email us at [email protected].
Listen to Life Kit on Apple Podcasts and Spotify , and sign up for our newsletter .
- Life Kit: Sustainability
- electric vehicles
- electric vehicle
- mass transit
More From Forbes
Tesla $25,000 model 2 needed, even stacked against model 3 price.
- Share to Facebook
- Share to Twitter
- Share to Linkedin
Future high-volume Tesla vehicles under wraps.
The Robotaxi is on the calendar. The Model 2 isn’t. That may say more about immediate strategic exigencies than anything else.
The Tesla Robotaxi announcement is set for August 8 per an April 5th tweet from CEO Elon Musk. But there has been no further word from Musk — other than denying a Reuters story — on the low-cost vehicle after he discussed it at the fourth quarter earnings conference call in January. At that time — a mere three months ago — he waxed eloquent about the upcoming car’s significance and manufacturing methodology. The car is often referred to by analysts as the Model 2 with a predicted price target of around $25,000. (More of what he had to say about the low-cost vehicle here .)
‘Debacle’ if canceled: One high-profile Tesla analyst said it would be a “debacle” if Tesla abandons the Model 2. “If robotaxis is viewed as the ‘magic model’ to replace Model 2 we would view this as a debacle negative for the Tesla story,” said Daniel Ives, an analyst at Wedbush Securities, on Thursday in a Tweet . He went on to say “it would be a risky gamble if Tesla moved away from the Model 2 and went straight to robotaxis” and that it is “crucial to deliver a Model 2 vehicle.” Indeed, Musk has been talking about this vehicle as a kind of Holy Grail since 2018 .
Skeptical about Model 2 retreat: In a research note containing a sub-heading of “But let’s not put narrative before the car” Morgan Stanley said this past week that “we have spoken with many investors who have interpreted the narrative shift away from Model 2 and towards robotaxi with great skepticism.” But the Morgan Stanley note did go on to say that current market realities may be forcing Tesla’s hand. Due to “a slowdown” in EVs, Tesla deliveries “are down nearly 9% YoY. Broader EV penetration has stalled in all key regions.” The note also cited “booming” hybrid and plug-in hybrid sales and vastly improved China competition. This may be forcing a “shift in Tesla’s strategic priorities,” the investment bank said.
Low-cost platform shared with Robotaxi? The Model 2 and Robotaxi may not be mutually exclusive. Musk in October called the next-gen vehicle "more conventional" and "utilitarian” which could apply to the hardware for both cars. “While development of the platform could be quite far along, it would be shared with the robotaxi,” Sam Fiorani, an analyst at AutoForecast Solutions LLC, told me in an email. But he added: “The margins on a low-cost model priced below the Model 3 will be tight and the current downward pressure on the Model 3's price would squeeze the life out of such a vehicle.”
The state of the Model 3 vs Model 2: A new Rear Wheel Drive Model 3 in inventory starts at about $35,000. With a $7,500 incentive distributed over the term of a 36-month lease and 10,000 miles per year and a $0 downpayment, that comes to $449 per month, not including upfront fees and taxes. A lease for a custom-order updated 2024 Model 3 (that sports design tweaks and interior improvements) with the same terms comes to $473 a month, according to the U.S. Tesla website. Note that the above numbers are based on a customized payment with $0 down payment. Hypothetically, if we’re talking about a Model 2 priced at, let’s say, between $25,000 and $28,000 with similar terms, a lease payment (or financing payments) would drop significantly and place the Model 2 clearly in a different market segment, making a Tesla accessible to many more Americans.
Best High-Yield Savings Accounts Of 2024
Best 5% interest savings accounts of 2024.
- Editorial Standards
- Reprints & Permissions
Putting on Airs: Lucid Needs to Sell More Cars, but How?
Lower prices and just getting the word out might help until the Gravity and an as-yet-unnamed midsize crossover arrive.
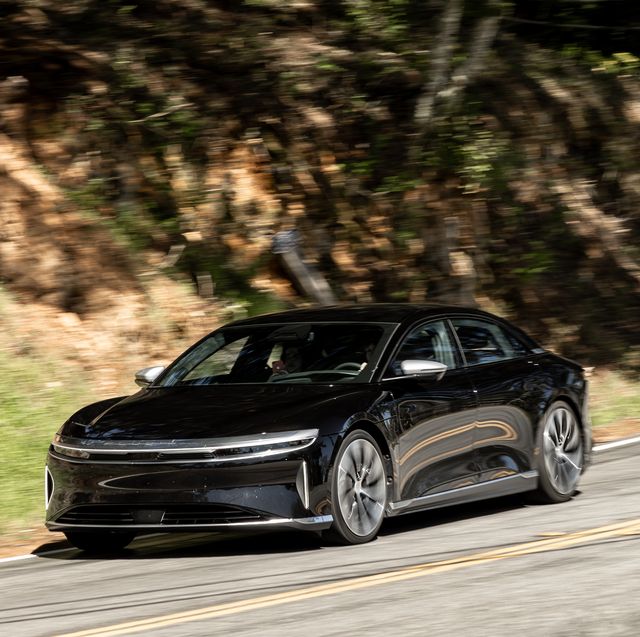
- Lucid wants to sell more of its Air sedans, so it's offering a sub-$70k model, a $649-a-month lease, and as much performance as you can afford.
- Lucid is on track to deliver the first Gravity SUVs later this year and even showed a midsize crossover under a tarp, saying it will arrive in 2026.
- But there is plenty of really good competition among all-electric luxury performance sedans, so it won't be exactly easy.
Lucid says it produced 8428 of its Air sedans in 2023 and delivered 6001 to customers. In the first quarter of this year, it lists 1748 cars produced and 1967 delivered globally. Deliveries were up nearly 40% year over year, the carmaker says, and up more than 13% quarter over quarter.
The competition is doing better, at least Tesla is. In that same first quarter of 2024, Tesla sold 168,500 vehicles, and 6000 of those were the Lucid Air’s main competitor, the Model S.
The rest of the competition is not doing much better than Lucid when it comes to moving luxury electric sedans out showroom doors. In that same first quarter of this year, Mercedes sold 1023 EQE electric sedans and 817 EQS EVs. Audi moved 776 e-tron GTs, Porsche 1247 Taycans, and BMW, well, AN doesn't break out how many BMW 7-Series sales were of the all-electric i7.
Cadillac was more successful than most in the segment, selling 5800 all-electric Lyrics.
So apart from the Tesla juggernaut, EV sales in the luxury/performance category are not what you’d call "huge."
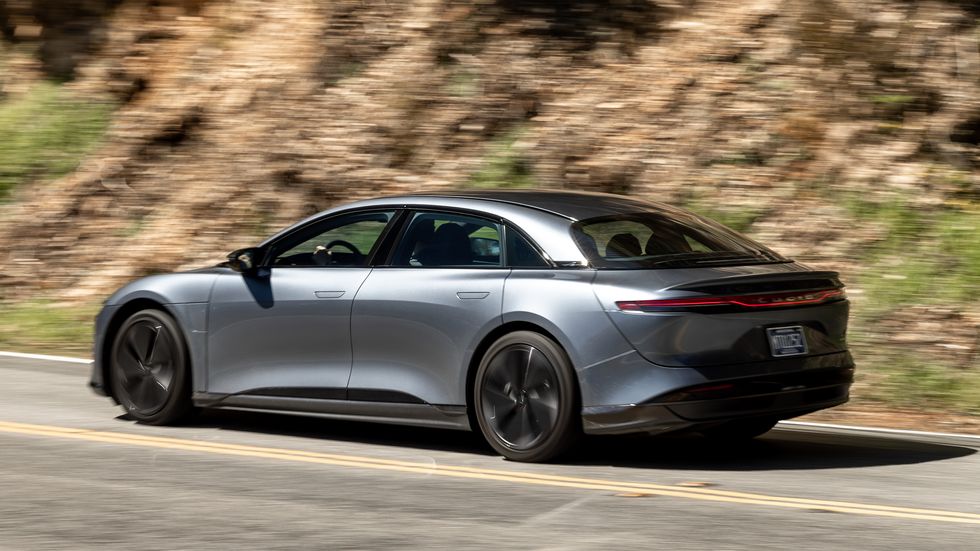
Lucid wants to give its sedan sales a boost while development continues on the big, beautiful Gravity all-electric SUV. Lucid also showed us a coming midsize crossover, sort of, without offering any details on the vehicle. But the midsize model should be relatively inexpensive, judging by the cheapest Air sedan, and it should share much of the same efficiencies of the rest of the Lucid line. But we don't know much about it yet.
For now, the company needs to sell more Airs, before the Air Apparent Lucid Gravity SUV comes online later this year. The market's crazy for crossovers, so once the Gravity hits showrooms, as has been the case when almost all luxury/performance carmakers started offering an SUV, sales should take off. Right?
Lucid is fully funded by the Saudi government's Public Investment Fund and a handful of other investors, so it's by no means desperate. It doesn't appear to be in financial trouble. And the factory is working overtime expanding and setting up for Gravity.
One thing it wants to do is get more people aware that it exists. Car enthusiasts know about Lucid, but much of the population apparently doesn’t. So they flew me and a bunch of my fellow press dopes up to company headquarters in Silicon Valley to show us around and let us drive all four models of the Lucid Air sedan.
The biggest news for 2024 is an improved Grand Touring model, one of the four Lucid Air models available, along with the Air Pure, Air Touring, and the rip-snortin’ Sapphire (more on that in a minute).
The 2024 Grand Touring can really tour, with an EPA range of 516 miles between charges, better than any production car in the world. For greater efficiency the model now gets a heat pump for heating and cooling instead of resistance heating and traditional air conditioning, Lucid says. It also receives enhancements to its motor design, battery chemistry, and thermal characteristics for greater efficiency, as well as faster charging—about up to 30% faster on Level 3 DC charging.
.css-ov6gys{font-family:Paralucent,Paralucent-roboto,Paralucent-local,Arial,sans-serif;font-size:1.5rem;line-height:1.1;margin:0rem;}@media(min-width: 48rem){.css-ov6gys{font-size:2.1875rem;line-height:1.2;}}@media(min-width: 64rem){.css-ov6gys{font-size:2.625rem;line-height:1.2;}}.css-ov6gys b,.css-ov6gys strong{font-family:inherit;font-weight:bold;}.css-ov6gys em,.css-ov6gys i{font-style:italic;font-family:inherit;} The Sapphire has 1234 hp and gets to 60 in 1.89 seconds, while maintaining 427 miles of EPA range.
"The Air Grand Touring is our longest-range car—in fact the longest-range EV available today—and is now further optimized with a multitude of powertrain updates, including the Air Sapphire heat pump," says Peter Rawlinson, CEO and CTO of Lucid. "The Air Grand Touring has retained its 516-mile range estimate, achieving this despite more-stringent EPA testing. More importantly, it delivers improved range and efficiency in a broader range of everyday, real-world conditions."
But it costs $109,900.
Fear not, Lucid's ace in the hole is that you can get a Lucid Air Pure, which almost no one will know is Lucid's entry-level model, for just $69,900. Now through April 30 they'll knock $5000 of that sticker "for vehicles available on-site at your local studio at the time of order." An 18-month lease is $649/month (with $3149 due at signing.)
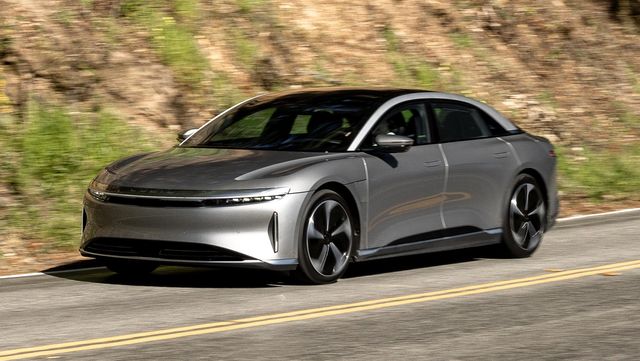
We got to drive all four Lucid Airs in the scenic coastal mountains between Silicon Valley and Santa Cruz, California, starting in the parking lot across the street from Alice's Restaurant made famous in—or maybe just named for—the Arlo Guthrie song.
I started out in the bargain Air Pure, getting lost immediately and winding up on Big Basin Way around Castle Rock, surrounded by enormous redwoods. The Pure has the smallest battery of the four models at 88 kWh, but it's good for 419 miles of EPA range, so I wasn't too worried.
On that twisty one-and-a-half-lane road the Pure hid its 4536 pounds well. The instant torque of its single 430-hp rear-drive electric motor pushed it right out of tight turn after tight turn all morning.
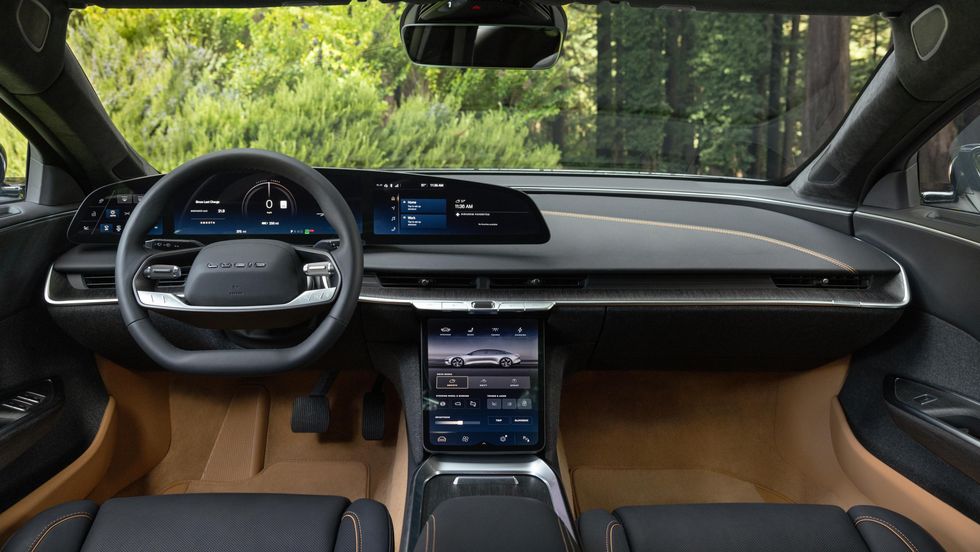
The best thing about the Pure is that the only way you can tell it's Lucid's cheapest model is by getting real close to the rear corner and squinting. "Air," it says in the smallest, most inconspicuous script ever put on a car.
I found Hwy. 9, then Skyline Blvd., then Alice's and jumped into the king grunion of all Lucids, the Sapphire . This one has three motors—two in back and one in front, balanced by an algorithm that helps stabilize the car in corners and deliver maximum torque to any wheel that has traction. Throw in independent regenerative braking and you almost can't screw up. Lucid uses the three electric motors in the Sapphire to really balance the car at its limit.
"This is the really fun thing about torque vectoring," says Esther Unti, senior manager of calibration and torque validation. "The limit's easy to find in a lot of cars, because they'll feel kind of linear until they understeer, or maybe like an S2k (Honda S2000) or something it'll feel linear until it oversteers. But with torque vectoring you can make it continue to follow the steering wheel regardless of whether you're on throttle or off throttle, and we can choose what we want it to do. We've tried to keep making it follow the steering wheel all the way up to the limit. It doesn't fall off and it doesn't feel like it starts to understeer, because it's just, linear."
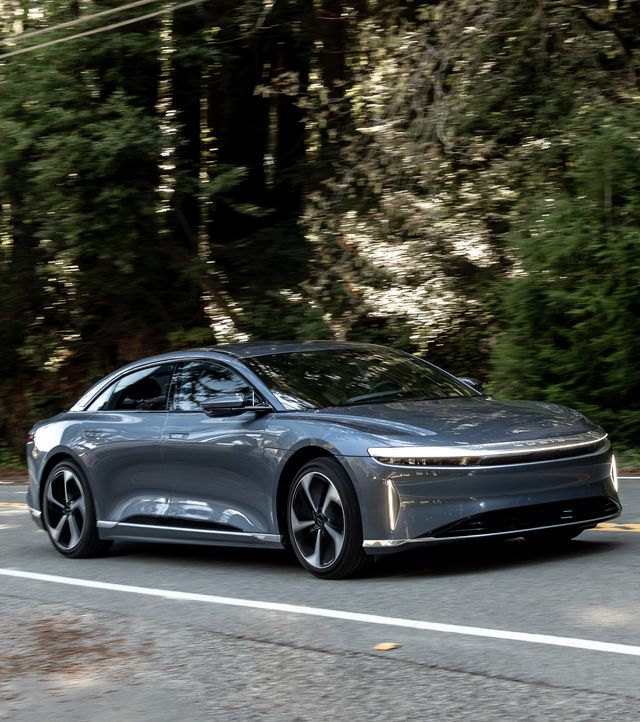
The Sapphire also has 1234 hp and gets to 60 mph in 1.89 seconds, while still maintaining 427 miles of EPA-certified range. I had been so satisfied with the performance of the Pure that I was all ready to say you don't need to spend the $249,000 for the Sapphire, until I went through the first curve in the Sapphire on La Honda Road (the correct route) and felt the grip, composure, and sure-footed glee with which this model attacked anything with a twist.
Whoooooo doggies!
You haven't driven a luxury sedan with this much precision and grip since your BMW M5. In any of the car's drive modes it is simply stunning. Is it worth a quarter million dollars? Heck yes it is, especially since I'm spending your money here.
I drove the Grand Touring and the Touring, too, and they were also good. Both have dual-motor AWD drivetrains, with the Grand Touring quickest to 60 mph by four tenths at 3.0 seconds and also winning in range with that well-documented 516 miles versus the Touring's 411 miles. The Grand Touring's also "only" $109,900 compared to the Touring's $77,900.
But the thing I came away with was that you'd be happy in any of these. The only constraint is budget. And for that you simply set priorities. The kids can go to state schools can't they?
Of course, you'd be happy in most of the competition, too. This is a great time to be shopping for an electric luxury performance sedan. And soon, for a luxury electric SUV. As Arlo Guthrie sang, "You can get anything you want…"
Which of the numerous luxury electric performance sedans do you like? Tell us below.

.css-1u92ux6:before{background-color:#ffffff;border:0 solid transparent;bottom:38%;color:#000;content:'';display:none;height:0.3125rem;position:absolute;right:0;width:100%;z-index:under;}@media(min-width: 40.625rem){.css-1u92ux6:before{height:0.625rem;}}@media(min-width: 64rem){.css-1u92ux6:before{bottom:25%;}} Lucid Motors
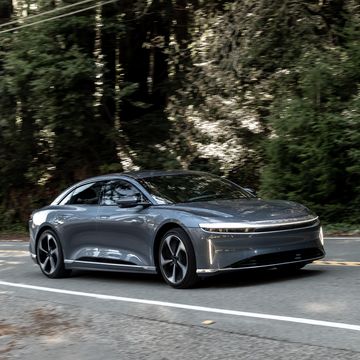
Are Price Cuts Paying Off for Lucid?
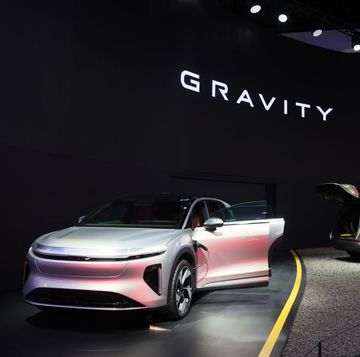
Here’s What Lucid Has in the Works Next
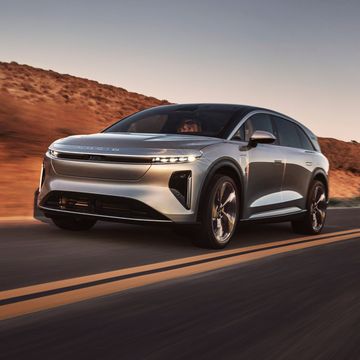
Gravity Will Make Lucid an Electric Power Player
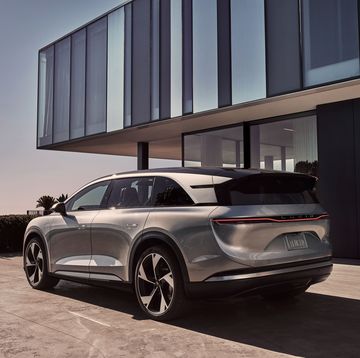
Gallery: 2025 Lucid Gravity Crossover SUV
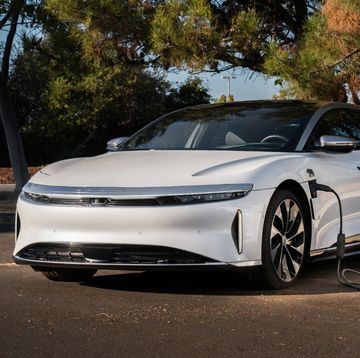
Lucid Will Be Able to Bail Out Other EVs
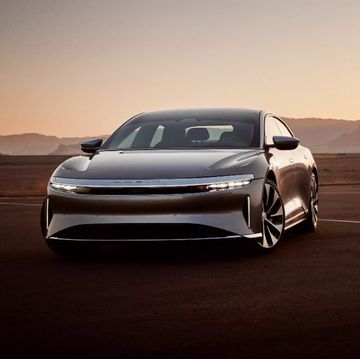
Here’s When Lucid Models Will Get Tesla Ports
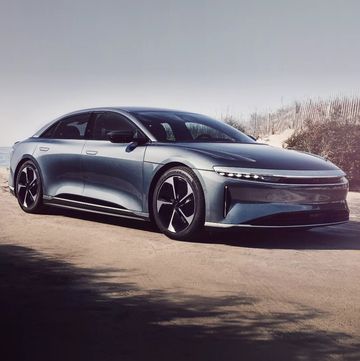
Can Lucid Turn These Numbers Around?
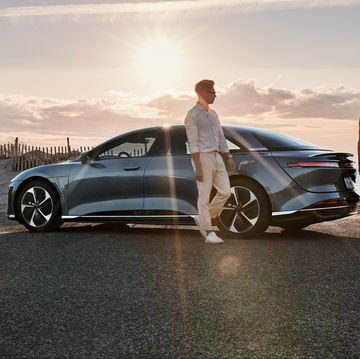
Here’s How Much the Cheapest Lucid Air Now Costs
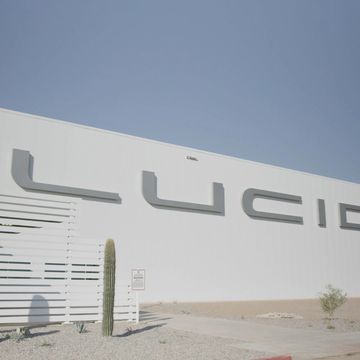
Lucid Cuts Ribbon on New Saudi Arabia Plant
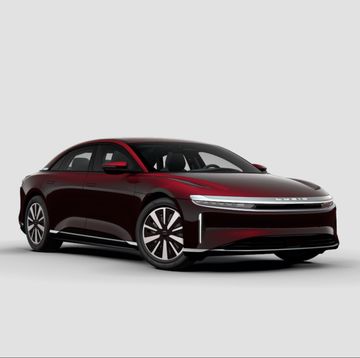
Here’s How Much Lucid Is Cutting Prices
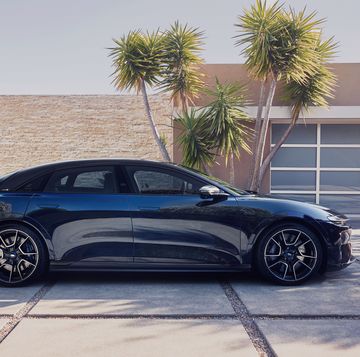
Gallery: 2024 Lucid Air Sapphire Photos
Review: 2025 Hyundai Ioniq 5 N enthusiast EV shows it's worth the wait
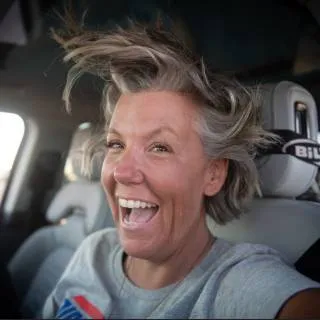
The good folks at Hyundai have scrambled my brain with the 2025 Ioniq 5 N, a devilish little electric compact crossover that might just be a motorsports game-changer.
Most electric vehicles are a blast to drive in a straight line, with incredible acceleration and accessible power. But their weight becomes a liability on the race track, and battery cooling can put a damper on the fun well before running through a charge.
Like many “sporty” versions of best-selling cars—think the Toyota Corolla GR or the Acura Integra Type S—the Ioniq 5 N gets more power, a wider stance, and upgraded tires. The car still has all the tech features we’ve come to expect in a Hyundai like automatic emergency braking, a driver-assistance system that helps ease fatigue on the highway trips, wireless Apple CarPlay and Android Auto, and a large digital gauge cluster and infotainment screen.
However, the company wanted to build an EV that would entice ICE enthusiasts over to the electron world. By and large, enthusiasts don’t care about tech features. Instead, we want emotion. We want to feel connected to the car, like the vehicle is an extension of ourselves. We want to feel joy and excitement and yes, just a touch of fear.
Whoo boy does this thing rock.
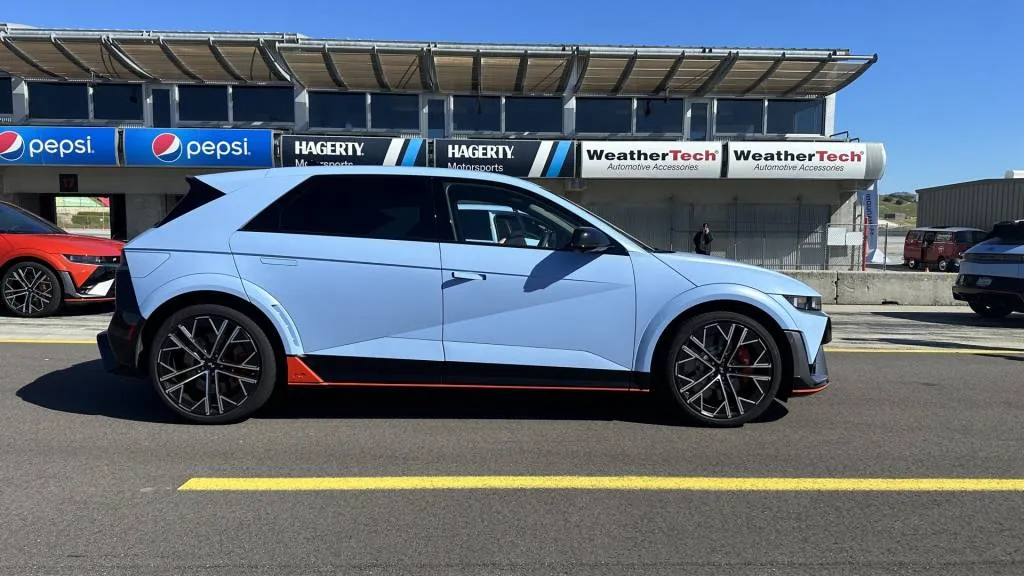
2025 Hyundai Ioniq 5 N - Emme Hall
Green Car Reports sent me out to Laguna Seca Raceway with five specific questions—in bold below—to answer. I spent the day amidst professional drivers and Hyundai engineers, dutifully fulfilling my assignment and wondering at the absurdity of hooning a ridiculously powerful car on a compelling track and getting paid for it. Y’all, it was a good day.
Does N Grin Boost put a grin on your face in the Ioniq 5 N? Did you use it on the track?
N Grin Boost is the equivalent to a go-fast gold coin in a video game. Press the red button labeled NGB on the steering wheel and the Ioniq 5 N gives you all 641 horsepower and 568 pound-feet of torque for 10 seconds. Drivers can do this as long as the battery is at a 30% or higher state of charge.
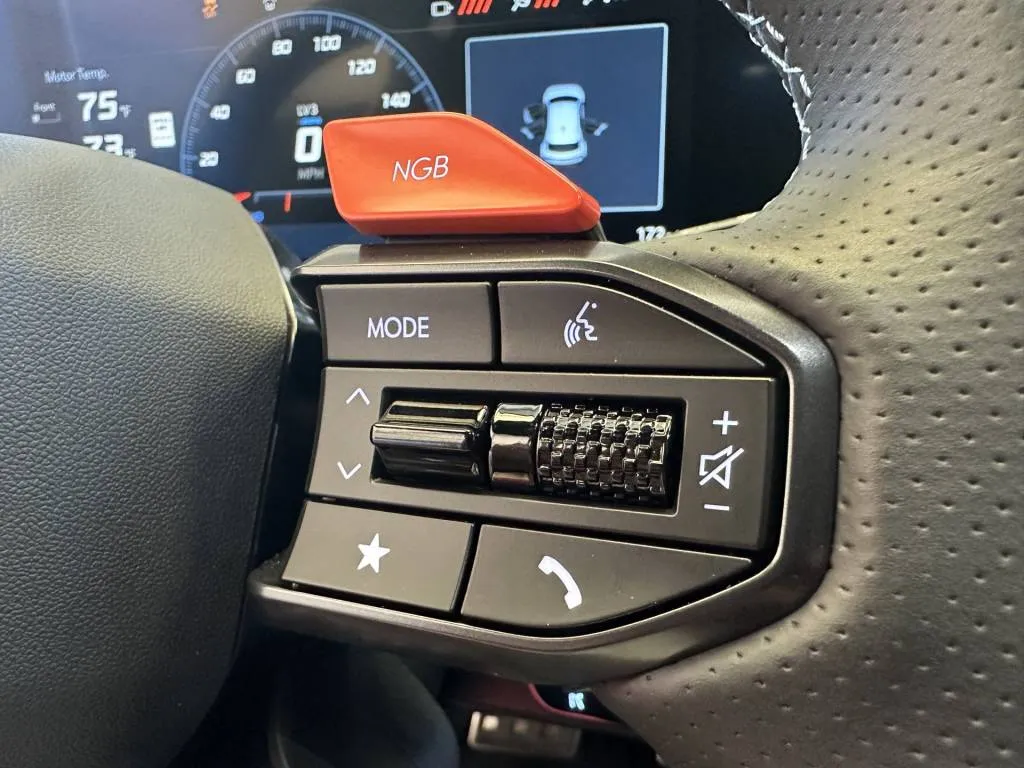
Laguna Seca Raceway is a complicated track with plenty of blind crests, tricky decreasing-radius turns and a 0.2-mile front straight. This straight, I figured, would be a good place to boost, and thus grin.
I’m not quite sure how fast I was going when I hit the button. I had just finagled my way out of turn 11, a 90-degree left-hand turn, so it couldn’t have been more than 35 or 40 miles per hour. I floored the accelerator, waited a few seconds and smacked my right thumb on that NGB button.
Did I grin? Yes. Did I also panic? Again, yes. I had been using one of Hyundai’s pre-programmed propulsion sounds—more on that in a minute—and as soon as the NGB kicked in, all went silent. It was like I was suddenly in a hyperspace vortex where time and physics meant nothing.
Space, however, was in full effect as I careened towards turn one, a blind crest that turns slightly left that has terrified me every single time I’ve been to this track. I risked a brief glimpse of my speedometer and saw 105 miles per hour.
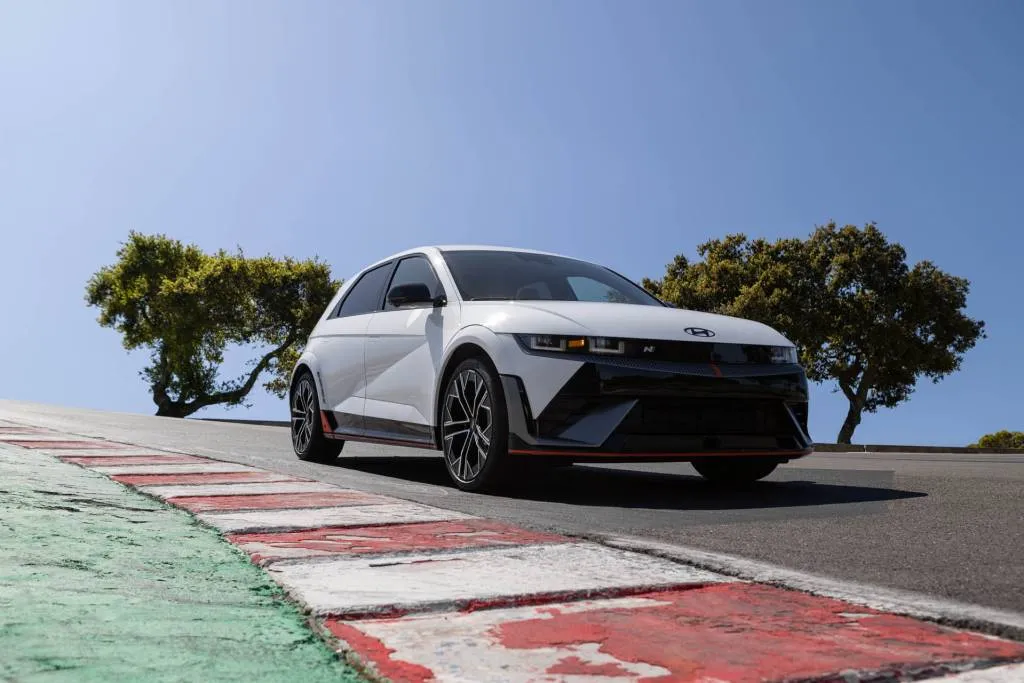
2025 Hyundai Ioniq 5 N
“This is fine,” I thought. “I’m fine, everything is fine.”
I managed to stay in the throttle over the crest without any kind of Code Brown moment, but the hysterical laughter that came out of me served as a reminder that I was indeed experiencing a tiny bit of fear. I got on the brakes hard and somehow managed to get through turn 2, the Andretti Hairpin, before NGB deactivated itself, the fake noises came back on and I was back to a slightly more manageable pace.
Hyundai later told me that NGB can be set to one of three audible sounds and doesn’t have to be silent. In retrospect it was kind of cool for the car to go quiet, as it was very clear something was happening. I just wasn’t necessarily prepared for it.
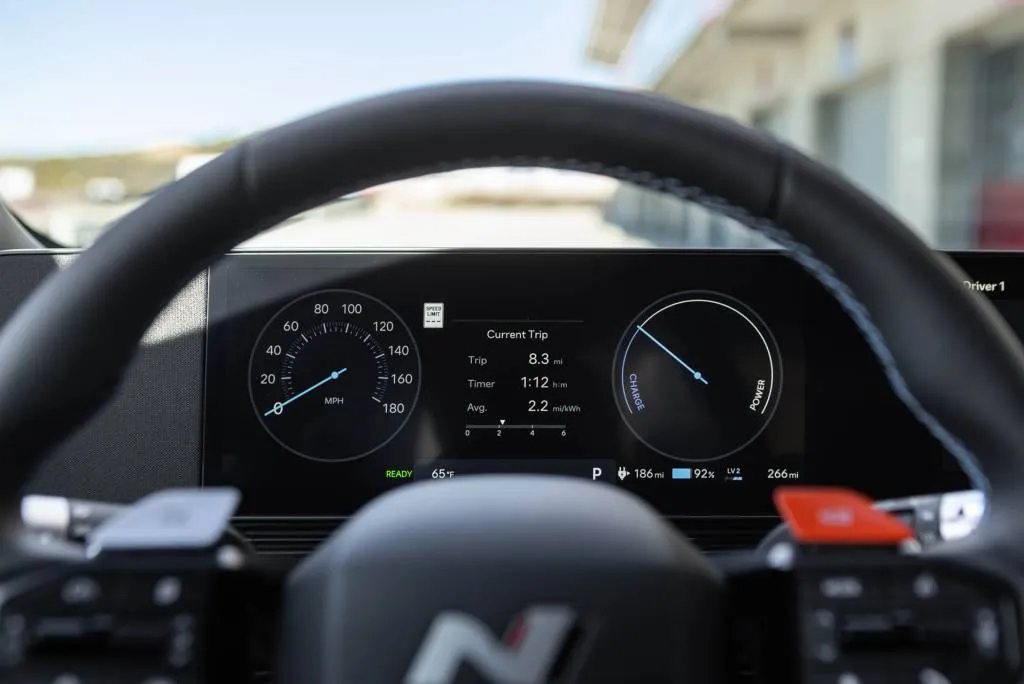
How do the fake gearshifts work in the Ioniq 5 N? Why did Hyundai choose this and did it ever consider a two-speed gearbox?
The Ioniq 5 N plays games with the driver with a simulated shiftable transmission, while it’s actually just running its motor up the rev range. Sure, I could keep it in Auto mode and let the two electric motors spin all the way up to 21,000 rpm, or I could let the computer in the N e-Shift feature manipulate my emotions a bit, keeping the motor—my perception of the motor—at a maximum of 7,750 rpm and divided into eight steps, making me feel more at one with the car.
Combined with the simulated sounds—and be patient, I’ll get there—shifting the car gave me a much more visceral experience. Auto mode is fine for toddling to the grocery store, but this is Laguna Seca, dammit. I wanted to feel like I was driving the car. I wanted to be in control.
The way the Hyundai engineers developed the simulated shifts is so simple it borders on genius and surely borrows from a driving simulator. When in manual mode, if I use the right paddle to upshift, the torque briefly drops then blips up, all in a nanosecond. From the driver seat it felt at minimum like a gentle push, but when I was on the throttle it was enough to jolt my head back a little, just like a real transmission would do when under load.
Coming into corners I could downshift and get some engine braking to help slow the car down, and put a few electrons back in the 84-kwh battery (more capacity than any other Ioniq 5). For science, I tried exiting turn 11 in fourth gear and the car protested with a noticeable lag, just like it would with an actual manual transmission.
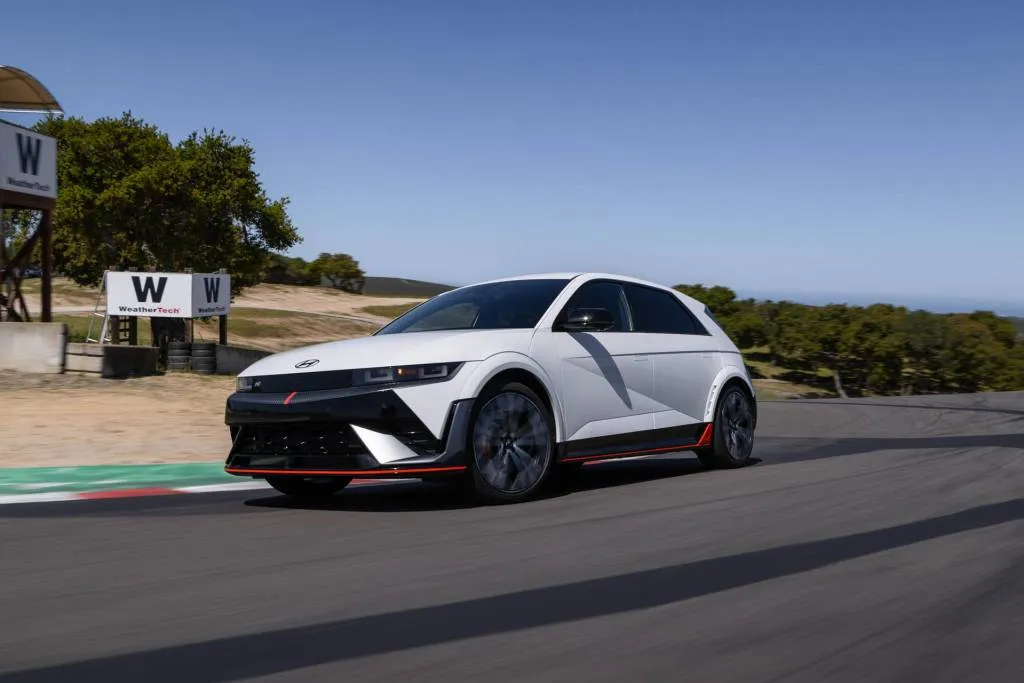
Hyundai could have gone the Porsche Taycan route and opted for a two-speed transmission, but again, it’s looking to build a bridge for ICE enthusiasts to cross over to The Democratic Republic of EVs. The company knows enthusiasts like manual transmissions and while this doesn’t have a clutch pedal, it at least gives the driver a modicum of control.
Joonwoo Park, Vice President N Brand Management Group, told me that a two-speed transmission would also add weight and cost. By eschewing a real, physical thing and merely adding a few lines of weightless code to its simple single-speed transmission, Hyundai adds lightness and helps keep the price of the Ioniq 5 N to $67,475 including $1,375 for destination.
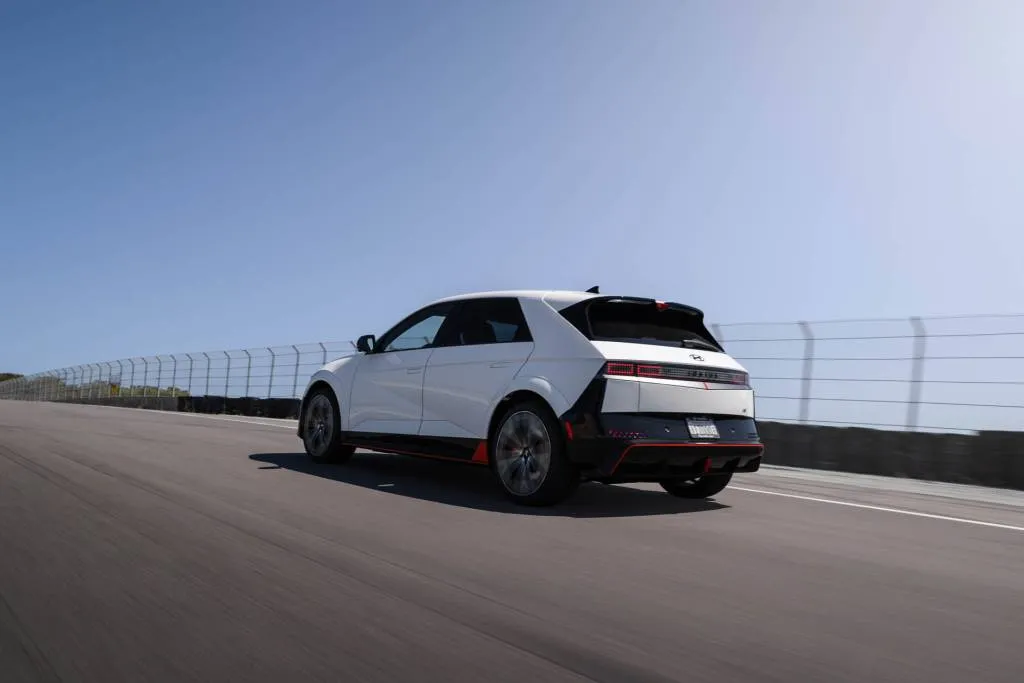
What about the Ioniq 5 N propulsion sounds themselves? What was the inspiration for this "simulated" part of its experience?
Okay, let’s talk about the sound! Hyundai has incorporated what it calls N Active Sound+ into the myriad choices drivers can make in the Ioniq N 5. Of course, you can have no sound, just the slight metallic whirr or the electric motors spinning up. That’s great for everyday driving but track shenanigans call for drivers to use all their senses, aural included.
The best sounding option by far is called Ignition. This is based on the 2.0-liter turbo four-cylinder engine found in the Elantra N, complete with lift-off pops and gurgles. My first session on the track was in silent mode with the single-speed gearbox doing its own thing. When I turned on the N e-Shift and the N Active Sound+, I got the physical motion of snapping off gears with the paddle shifters and the audible cues of a throaty little engine. Suddenly I was much more invested in the experience, and my time shows it. Sure, I’m no Max Verstappen, but my second session went by much quicker than the first, and it was much more fun to boot.
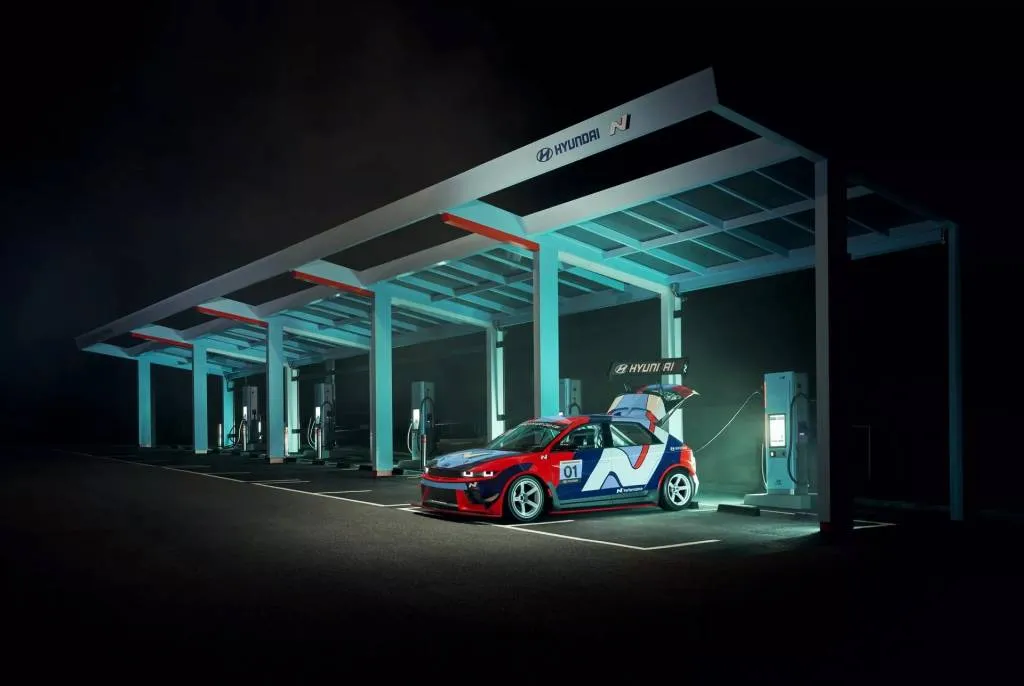
2024 Hyundai Ioniq 5 N eN1 Cup race car
The sound is pumped into the car from eight speakers, but also released to the unsuspecting pedestrian through two external speakers. Park said that those participating in the new eN1 Cup racing series in South Korea with the Ioniq 5 N may one day get to choose their own bespoke sound, so fans know who is coming around that corner and on to the straight.
I think I’d want mine to sound like a Mötley Crue guitar riff, but I digress.
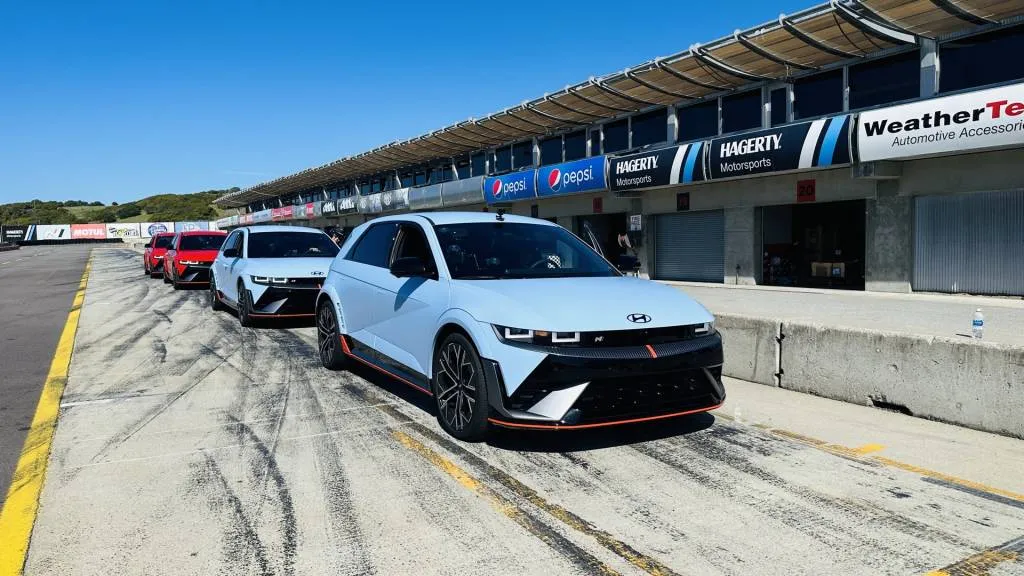
Preconditioning is as much about getting the most track time as it is getting the quickest charge. How does this all work in the 2025 Hyundai Ioniq 5?
Of course, EVs have a few disadvantages when it comes to running hot and heavy on the track. Sure, an ICE vehicle will likely need more fuel during a day of track driving, but it’s a task completed in five minutes or less. With an EV, there are so many other components that come into play to make sure the batteries can deliver the most power and recharge quickly.
First, there is power delivery. The battery in the Ioniq 5 N can be set for a sprint race with an optimal battery temperature of 86 to 104 degrees Fahrenheit or an endurance race, where the temperature should be a bit cooler, between 68 and 86 degrees or so. Keep in mind that these numbers are for optimal performance, not range. During my time on the track I lost 10% of my charge in each of my two endurance sessions and the battery temperature stayed around 70 degrees. I used 16% of my battery juice in my one sprint session ending with a battery temperature of 93 degrees.
The Ioniq 5 N has triple the amount of cooling surfaces as the regular Ioniq 5, a cooling element inside the battery and its own separate radiator as well. Further, the computer can tell the driver how long it will take to reach optimal temperature, so she knows just how long before the next track session is a go.
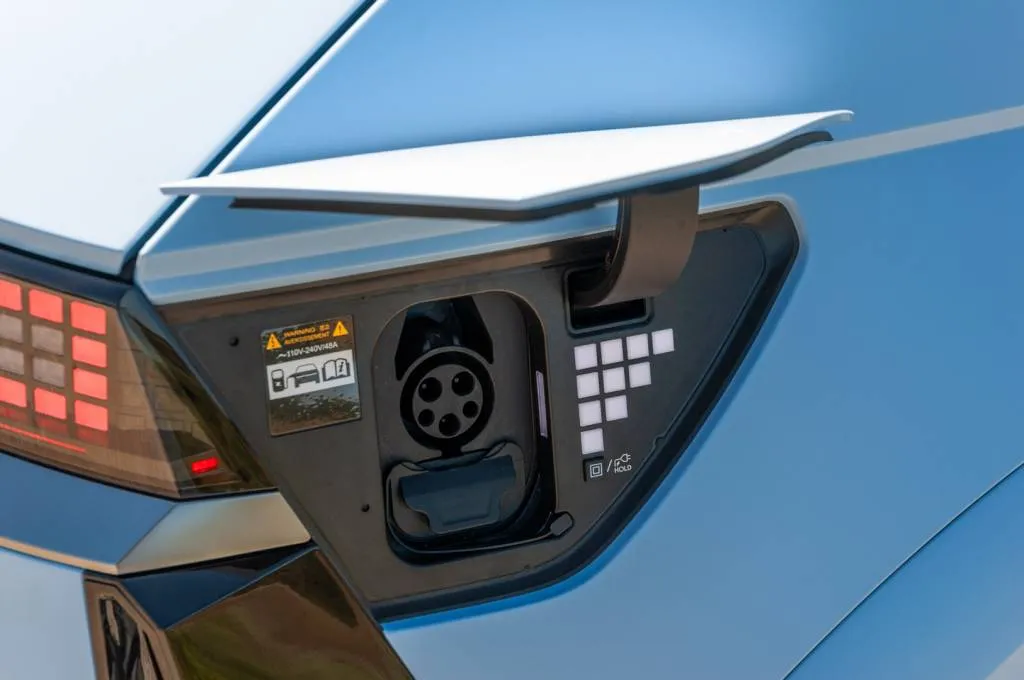
When it comes to charging, the Ioniq 5 N can fast-charge at a peak of about 235 kw thanks to its 800-volt architecture. However, anyone with any experience with an EV knows that rate is not guaranteed. Not only does the charger need to be reliable enough to deliver the power; the battery pack needs to be ready to accept it. Like most EVs, the Ioniq 5 N can condition the batteries for charging, getting temperatures in that sweet charging spot between 68 and 86 degrees.
Hyundai has installed 10 high-speed chargers at the Inje Speedway in South Korea to support its new eN1 Cup racing series. It also hopes to put chargers at tracks around the world including the famous Nurburgring, where the company says the Ioniq 5 N can do two laps flat-out of the nearly 13-mile track in Endurance mode.
As for charging at Laguna Seca, Hyundai had to hire a few trucks with generators to keep the electrons flowing. There are Tesla Superchargers but the Ioniq 5 N does not have NACS capability, nor is the station a Magic Dock station. Nuts.
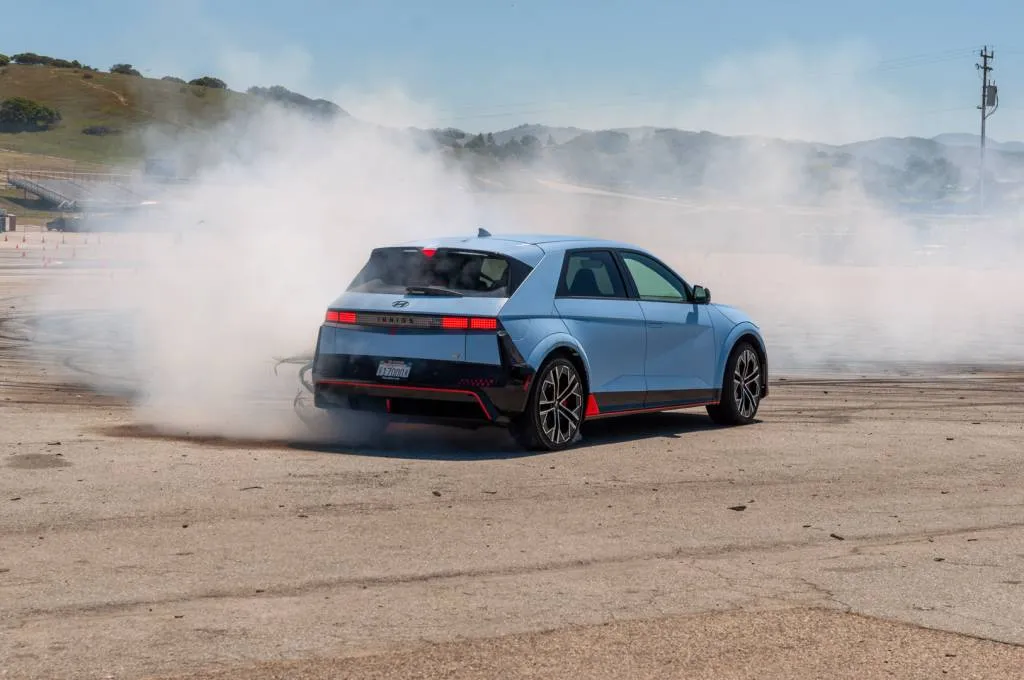
Overall, does the Ioniq 5 N compare to the EV6 GT in driving thrills—and in driving range?
Although the Kia EV6 GT might be built on the same platform as the Ioniq 5 N, the two are hardly comparable. Don’t get me wrong, the EV6 can generate 576 horsepower and 545 pound-feet of torque from its two electric motors—close—and it shares a few features with the Ioniq 5 N like an electronic limited-slip differential and a drift mode, but not its larger battery and intensified pack cooling. At the end of the day the EV6 GT is a quick car that can carve a canyon or two. It’s not a track car.
The EV6 GT doesn’t have an e-Shift feature nor deliver any audible cues, both of which enhance the Ioniq 5 N to the, ehem, nth degree. The Hyundai also has distinct settings for the motors, the steering, the electronic limited-slip differential, suspension and steering feel and the driver can push close to 100-percent of the torque to the rear wheels if he so desires.
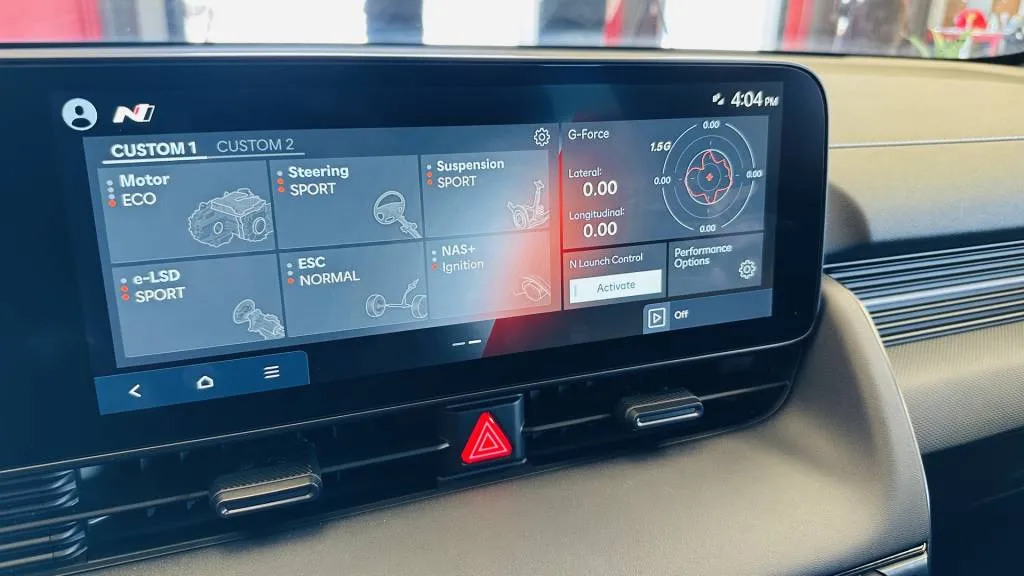
There are even two different kinds of brake regeneration: I-pedal with your standard levels of regen or N pedal, which is meant more for weight transfer. Select this setting and the 0.36g of regen not only slows the car before a turn, but really gets the weight moved to the front, helping the Pirelli P Zero tires grip through the turns. Yeah, you’ll hear some squealing but trust me, the grip is there. The EV6 GT with its less sticky Goodyear Eagle F1 shoes just can’t compare.
As for driving range, well, that’s a tough one. I got to sample the Ioniq 5 N on public roads, but not for an extended amount of time. Hyundai says the 84-kwh battery is good for 221 miles of range—less, of course, while hooning on a track. Meanwhile the 77.4 kWh battery in the Kia EV6 GT can eke out 206 miles. Again, I would expect it to be difficult to hit that EPA number if you exploit the GT’s power at every green light.
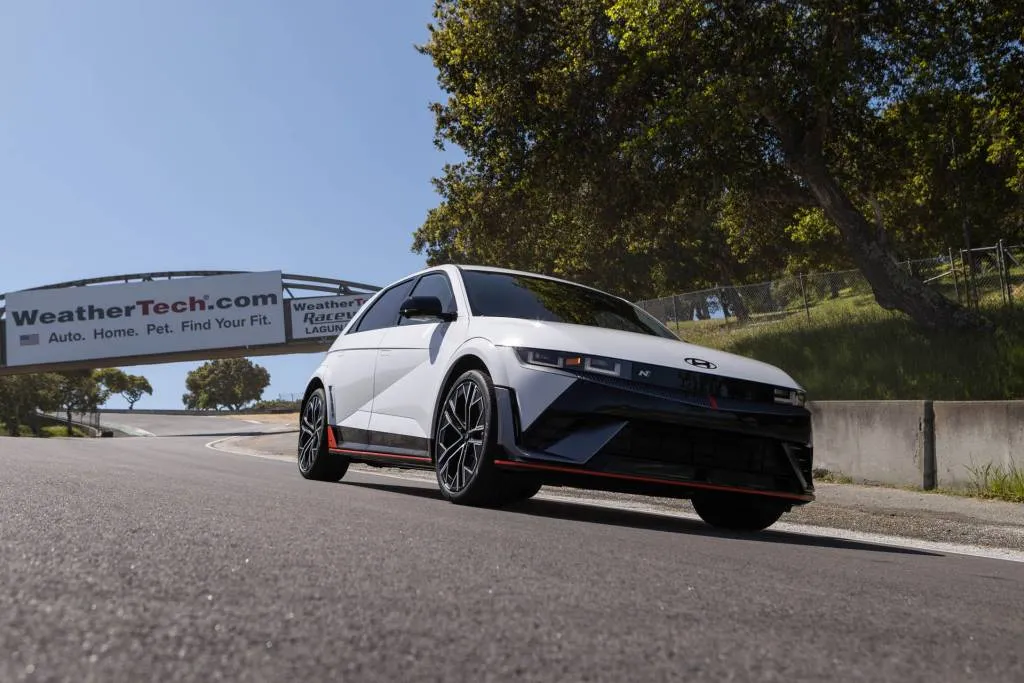
Hyundai Ioniq 5 N summary: Driving joy
By the time I left Laguna Seca I was worn out. I had pushed through the fearsome turn 1 at triple digits, bothered Hyundai engineers with technical questions and loved every second of it. While the 2025 Ioniq 5 N might be a rolling computer with myriad complicated settings, it produced more emotion and amusement than I thought possible from an electric vehicle.
This isn’t a car about saving the world with a green powertrain. It isn’t about making a commute safer with advanced driver’s aids or even about pushing the boundaries of all-electric range. No, the Ioniq 5 N is about driving joy. And I’m here for it.
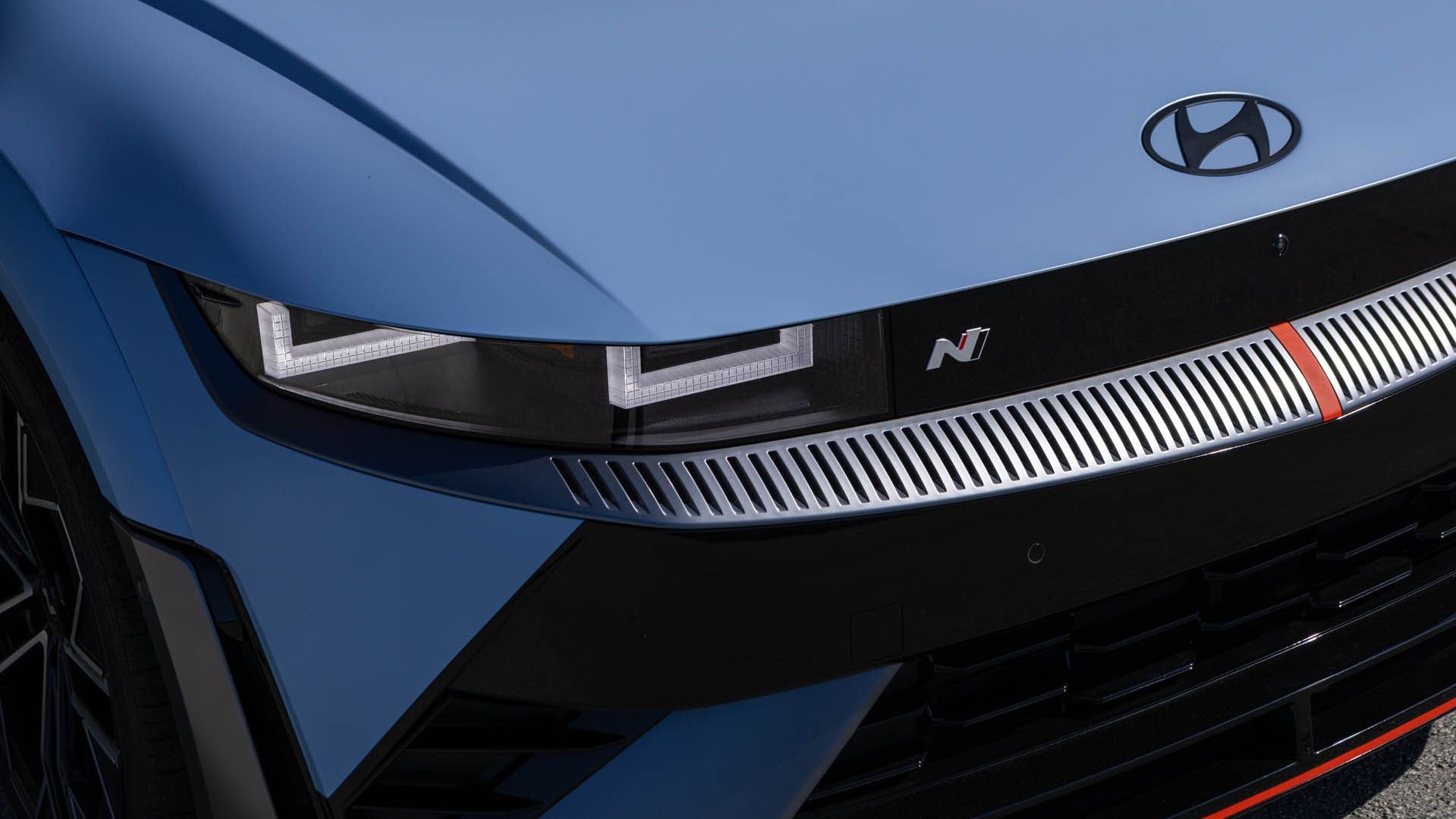
Contribute:

How it Works:
- 1 Select your vehicle
- 2 Provide your contact information
- 3 Choose dealers to contact you
People who read this, also read:
- 2024 GMC Sierra EV boasts 440-mile range, CrabWalk, lower price
- Maserati reveals US-bound luxury electric convertible
- 2025 Mercedes-Benz eSprinter adds smaller battery, shorter wheelbase
- Rivian grades chargers, China's battery problem, EV ownership cost: Today’s Car News
Share This Article:
Connect with the editor:, follow us today:, green car reports newsletter.
Sign up to get the latest green car and environmental news, delivered to your inbox daily!
I agree to receive emails from Green Car Reports. I understand that I can unsubscribe at any time. Privacy Policy.
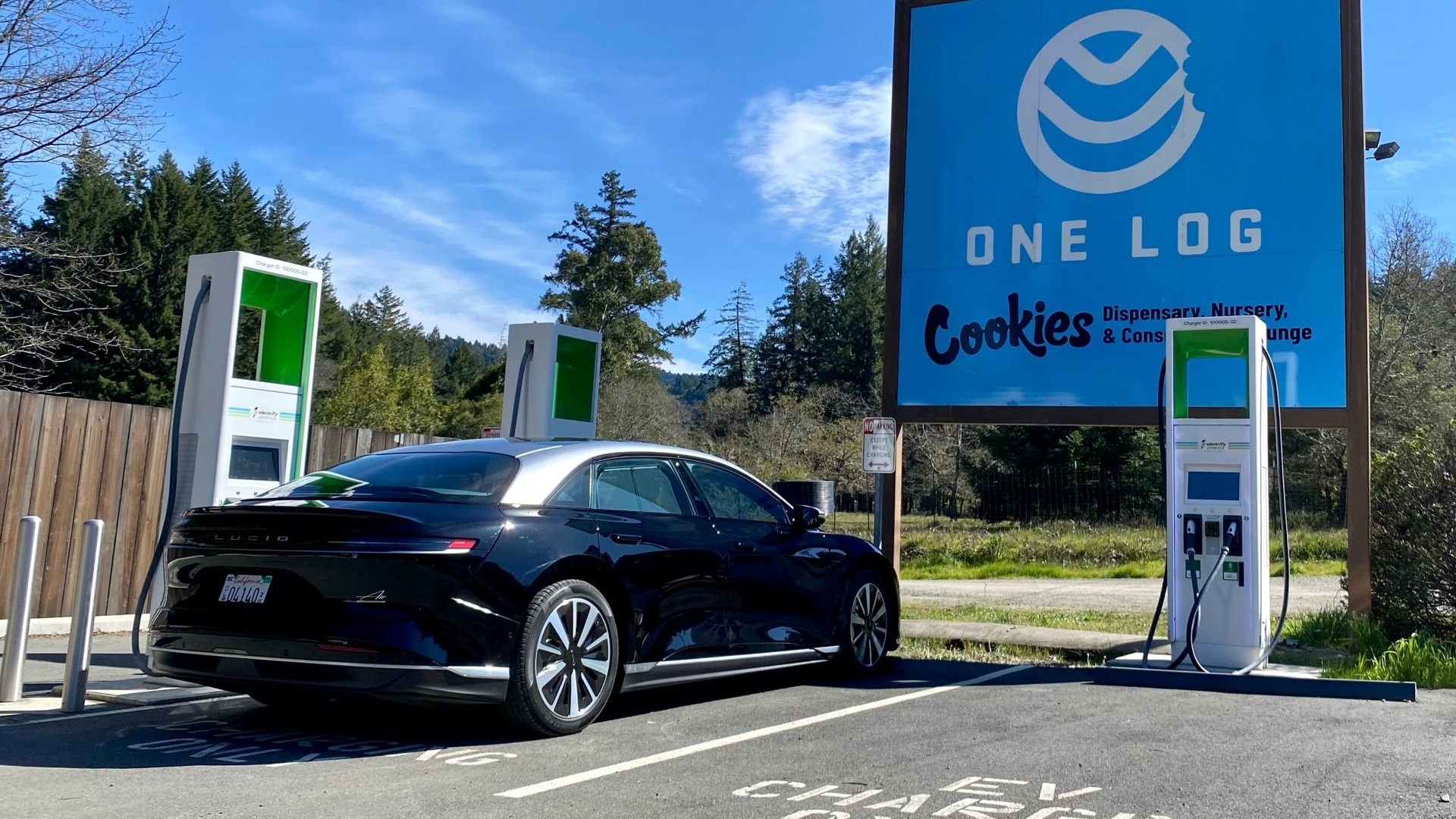
Lucid adds a heat pump and subtracts charging time for its range-leading Air electric sedan. Mercedes-Benz might not see what Ram sees in a plug-in range-extended vehicle. And why is each EV still losing thousands to full-line automakers? This and more, here at Green Car Reports. The 2024 Lucid Air Grand Touring is now being delivered, and once again it hits a 516-mile EPA range—the best of any EV. But with the addition of a head pump, improved automatic preconditioning, and up to 30% faster DC charging, it’s likely to cut the time spent at road-trip charging stops even more...
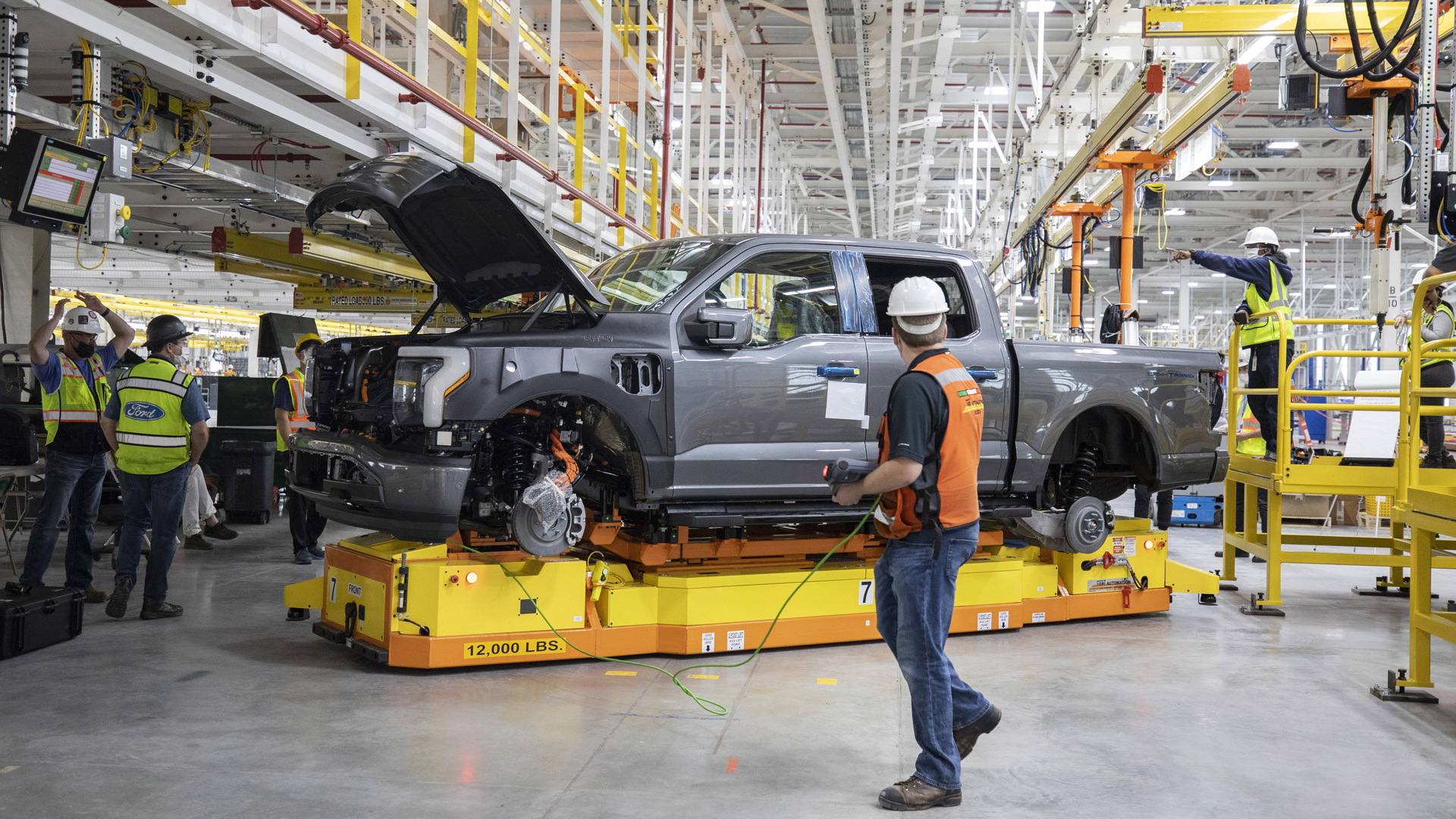
A recent analysis suggests automakers are still losing thousands on each EV sold—perhaps even on programs that have targeted profitability.
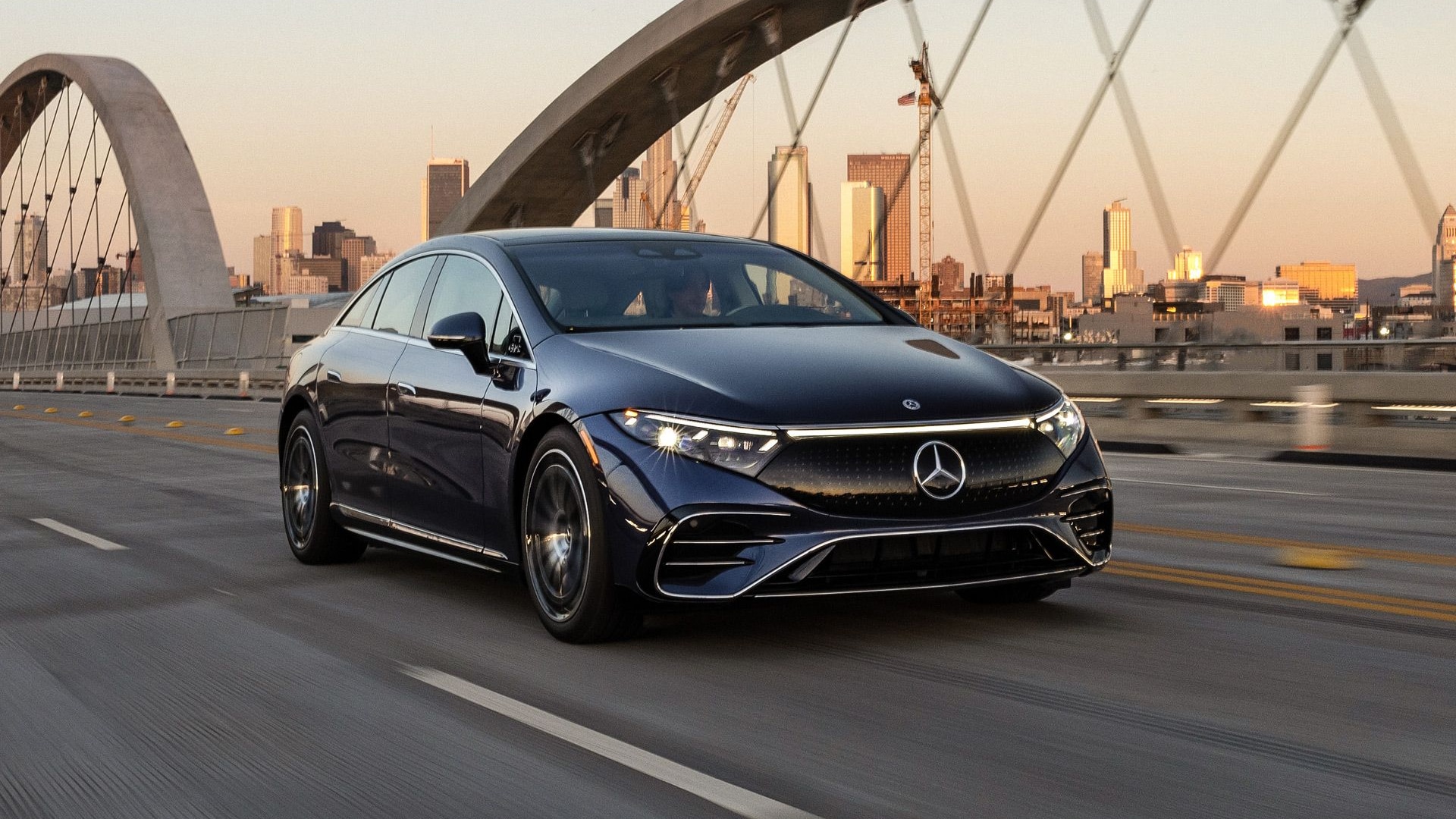
Series hybrids with vastly downsized engines simply don't make the cut, especially for cost reasons, Mercedes reportedly decided.
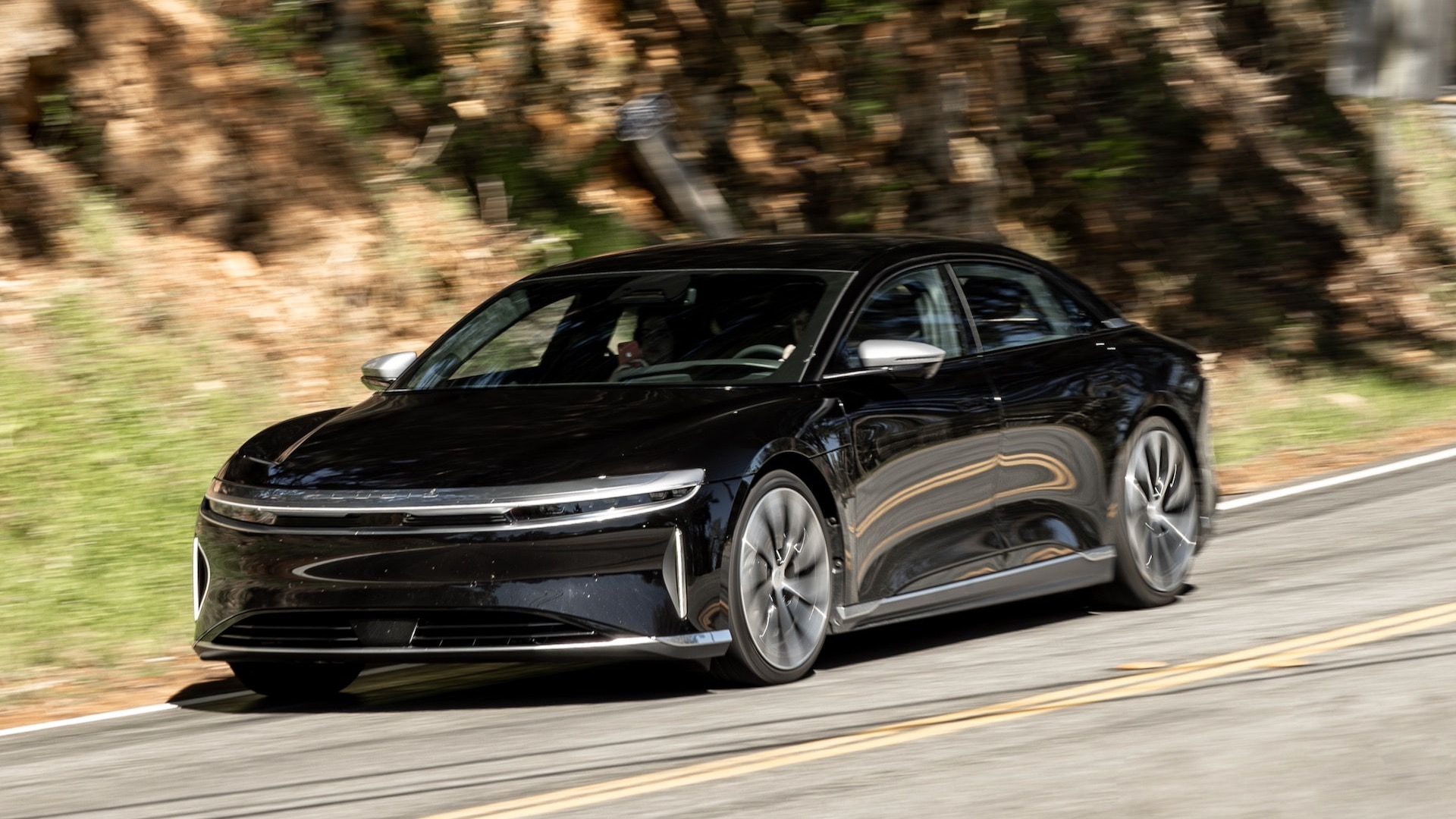
The Grand Touring also preserves its 516-mile range rating despite more stringent testing, Lucid claims.
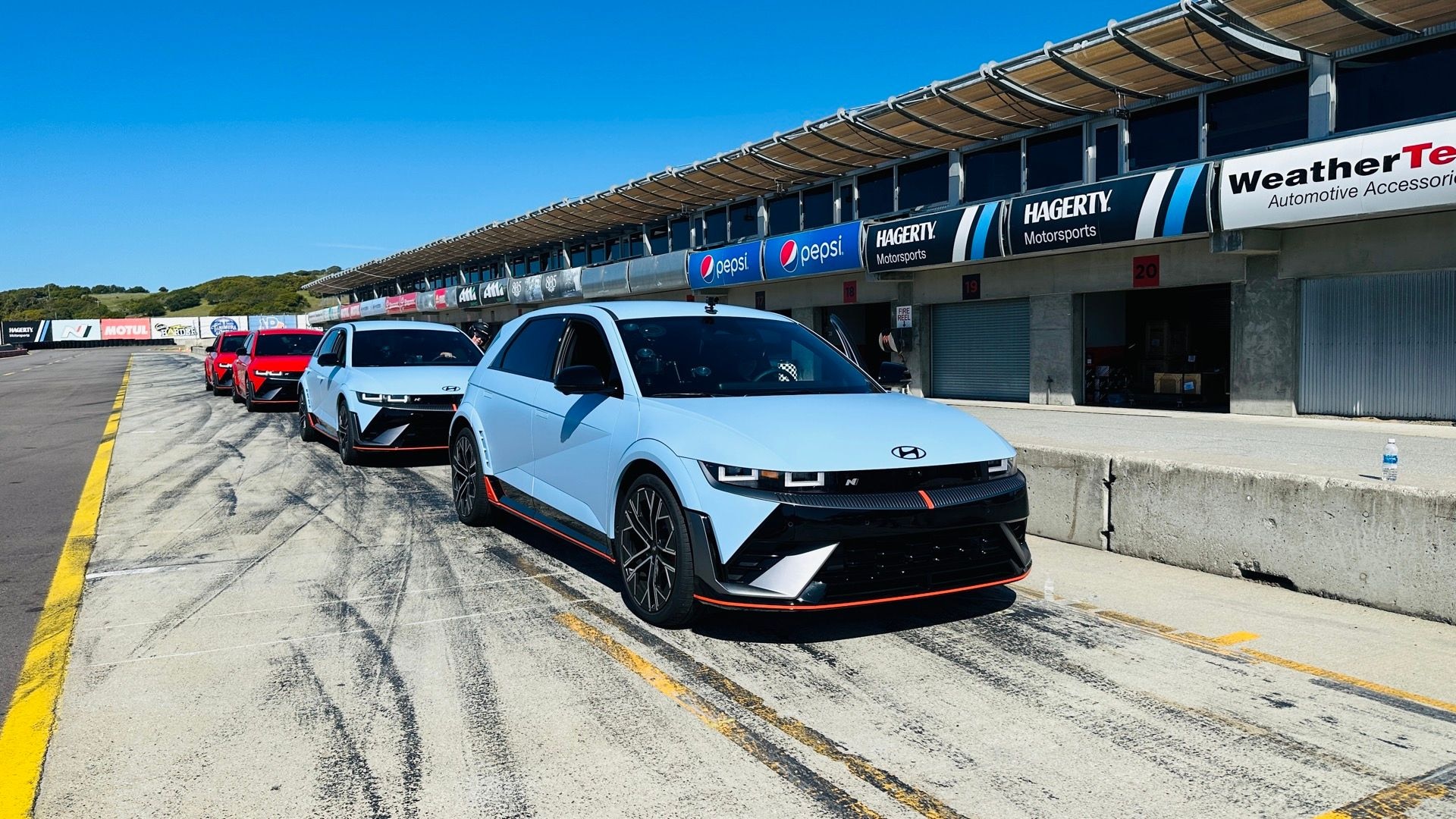
Maserati might have the first U.S. electric convertible in years. Mercedes adds lower-priced versions of its eSprinter. GMC sets the price, range, and more for the arrival of its Sierra EV pickup. And we look at how the Hyundai Ioniq 5 N gamifies its interface to actually make driving more...
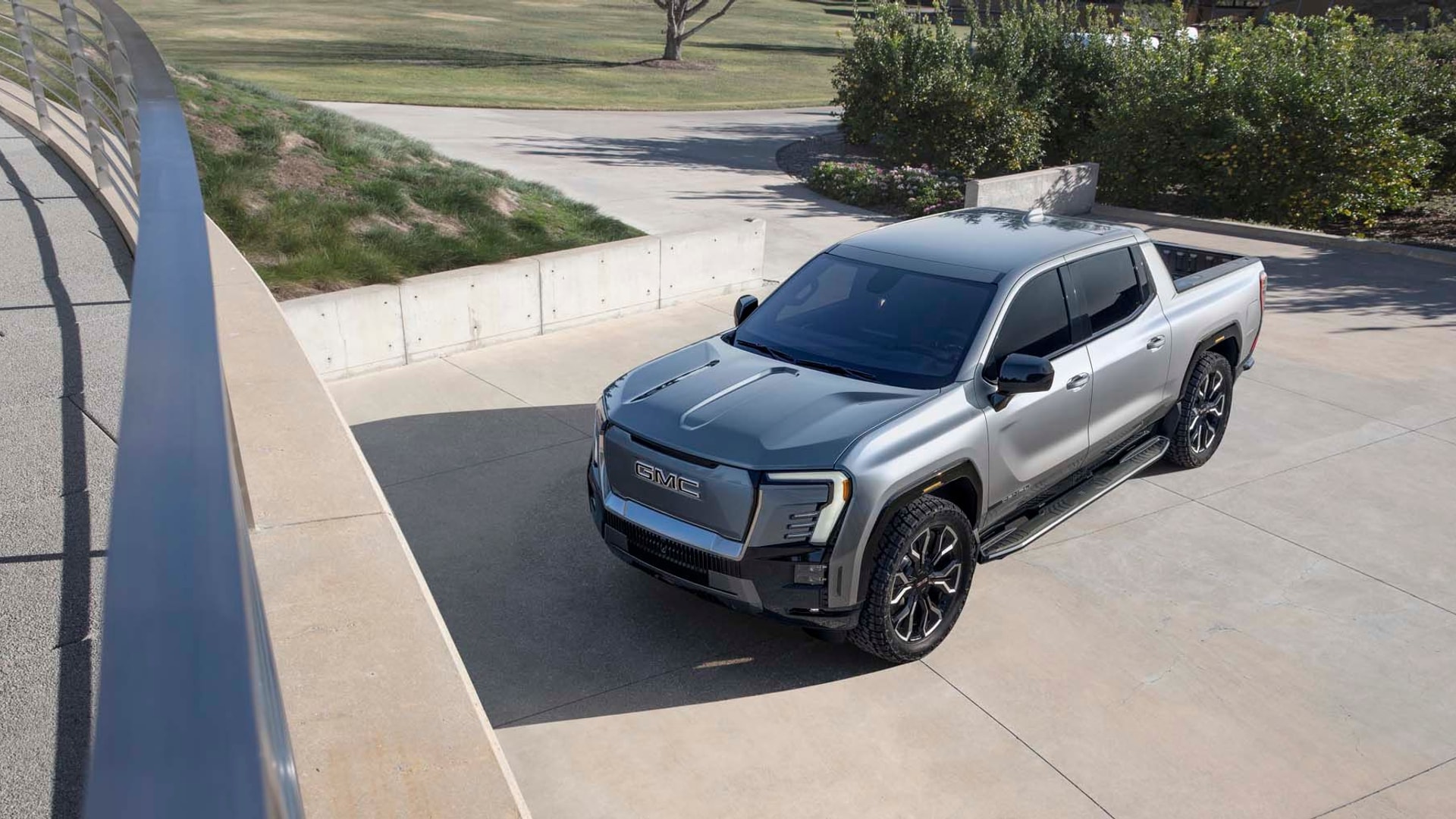
The top-trim Denali version of the Sierra EV electric pickup arrives in summer 2024 with a lower price and more range than originally outlined.
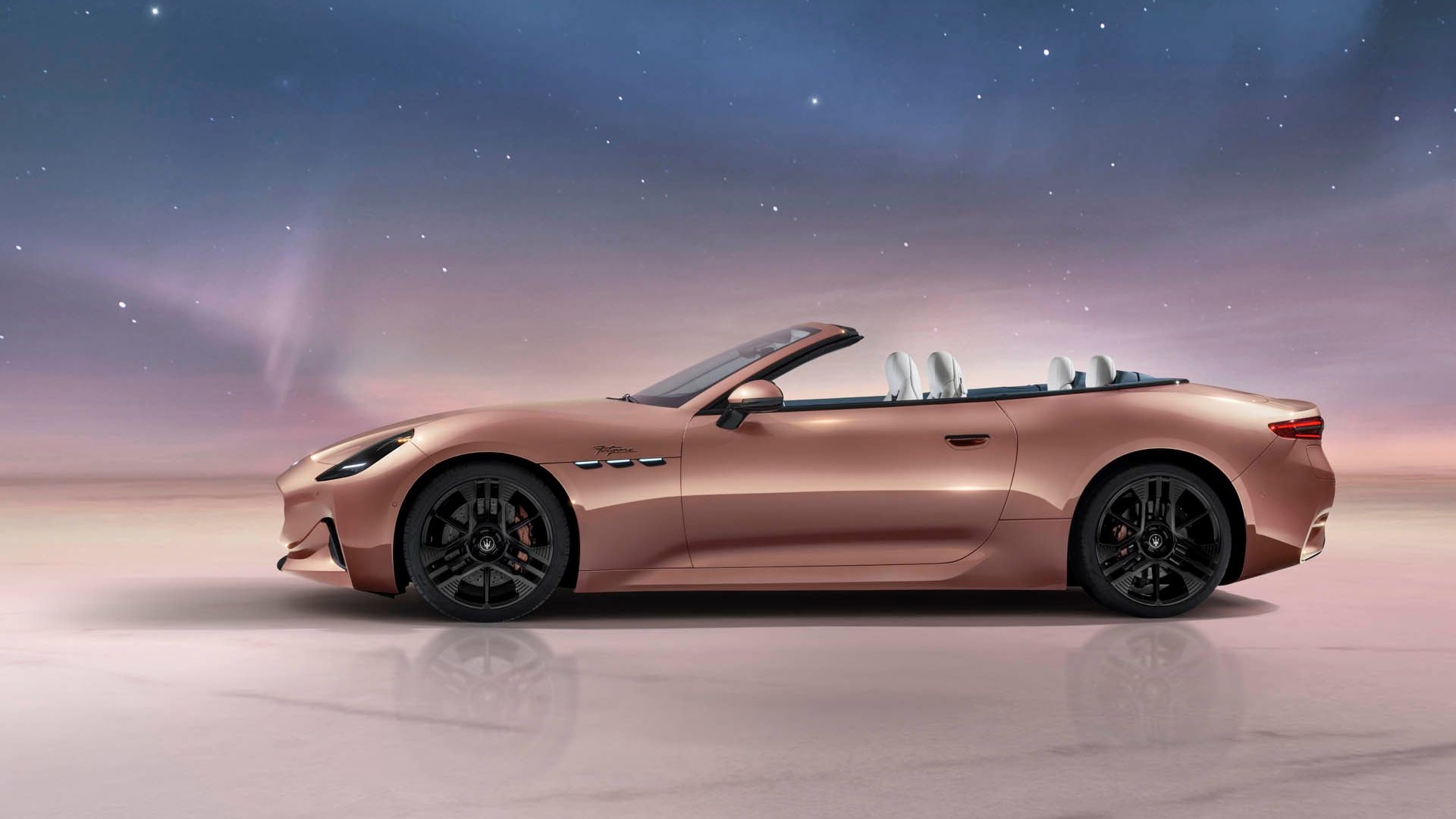
The GranTurismo Folgore might be the first fully electric convertible to arrive in the U.S. in years.
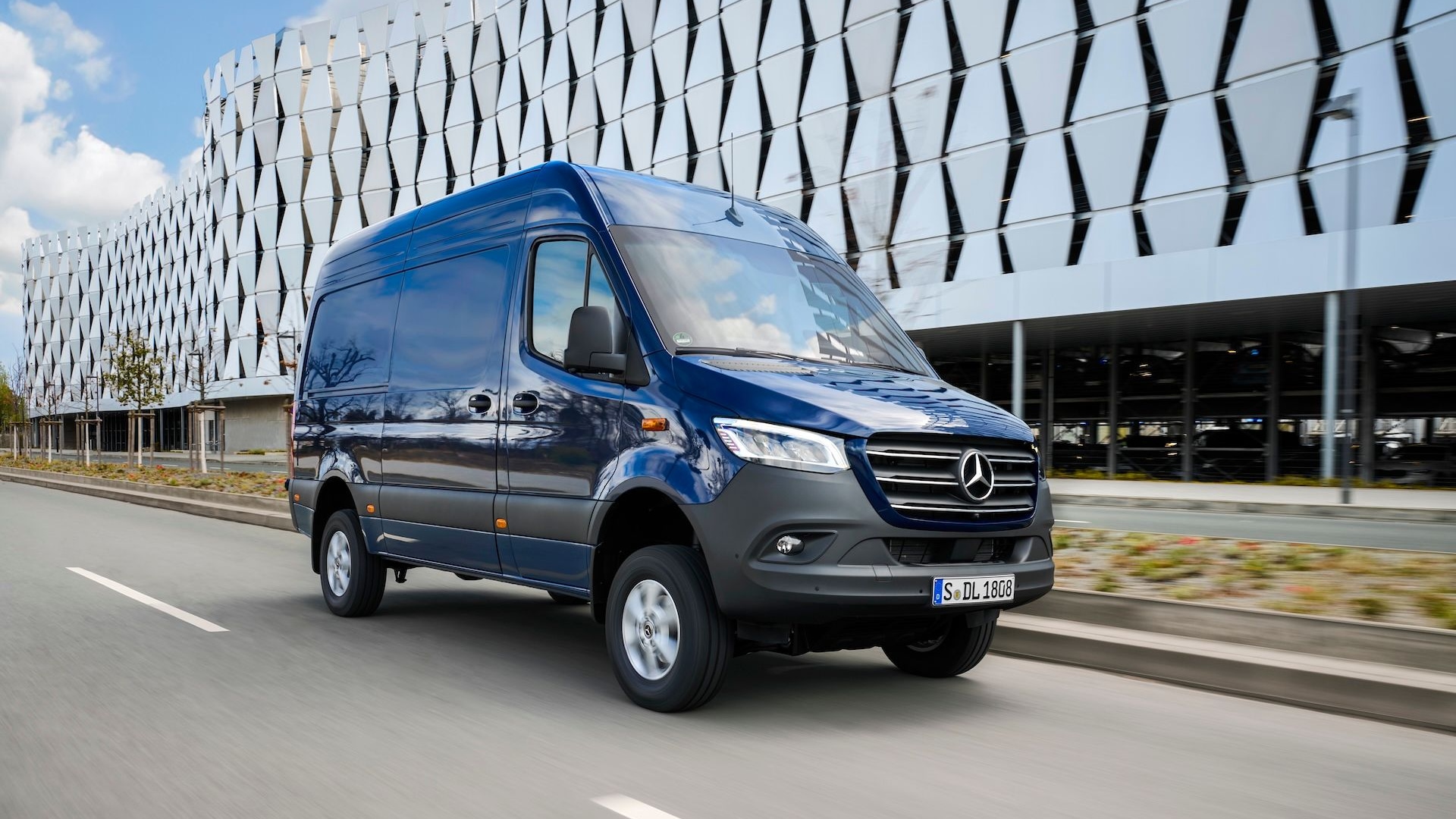
A smaller, more affordable version of the Mercedes electric van gives fleets more options to fit their needs.
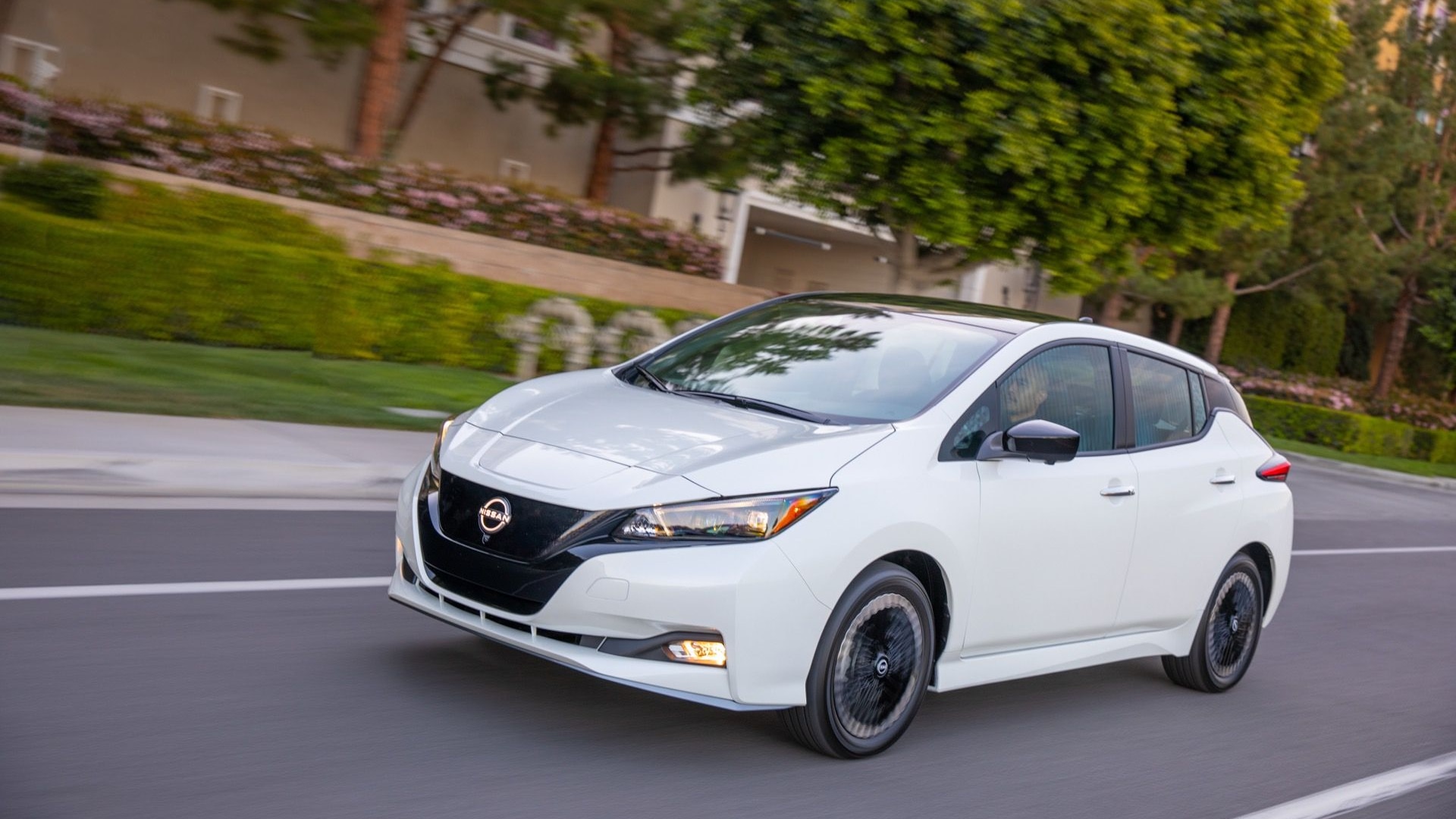
China is making too many EV batteries. Rivian is applying real-world letter grades to EV fast-chargers for smoother route planning. And despite depreciation woes, many EVs still pay back big over five years of ownership. This and more, here at Green Car Reports. Rivian has announced that it’s...
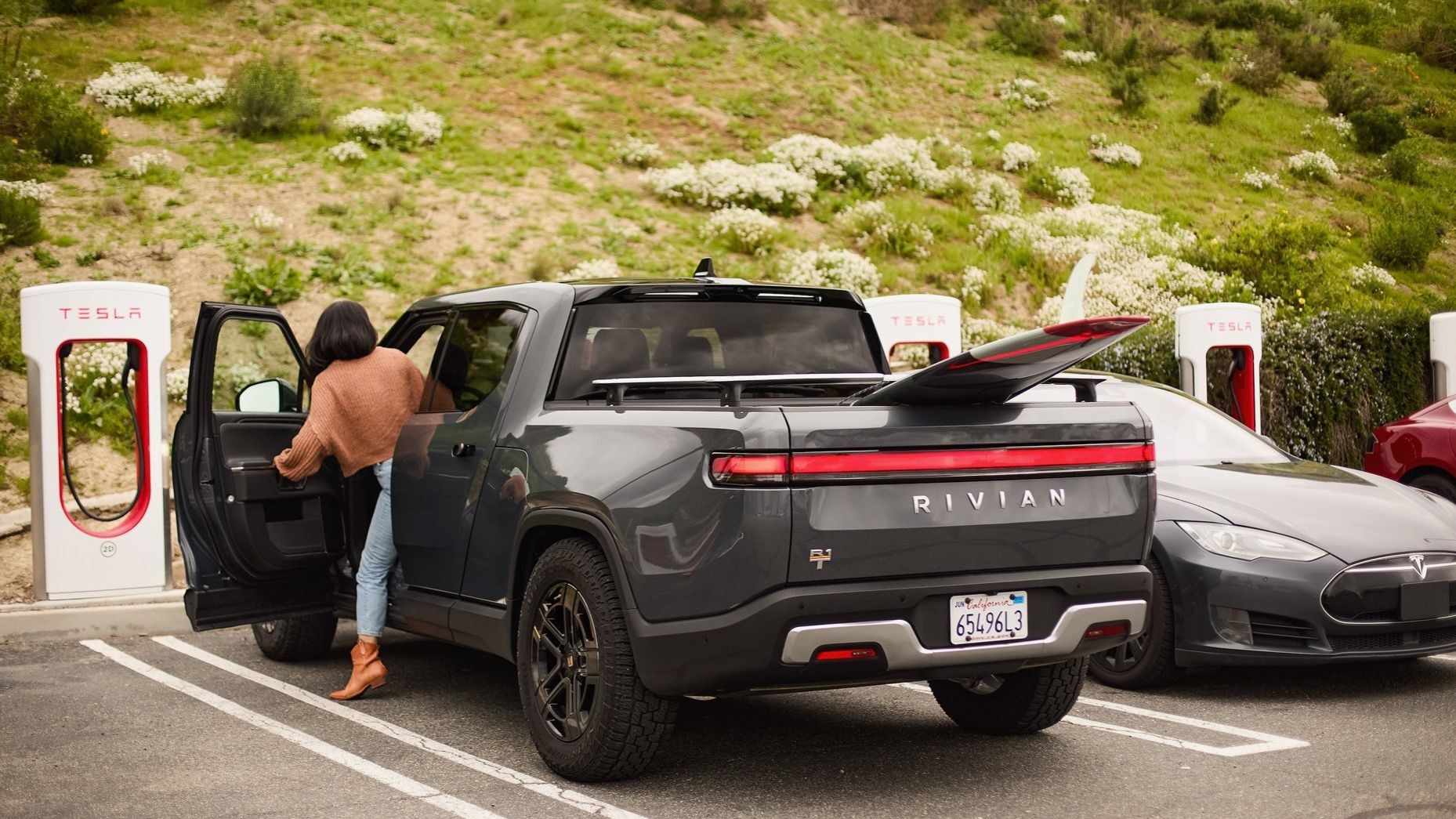
Every DC fast charger Rivian uses for route planning will be given a reliability grade from A (best) to F (worst).
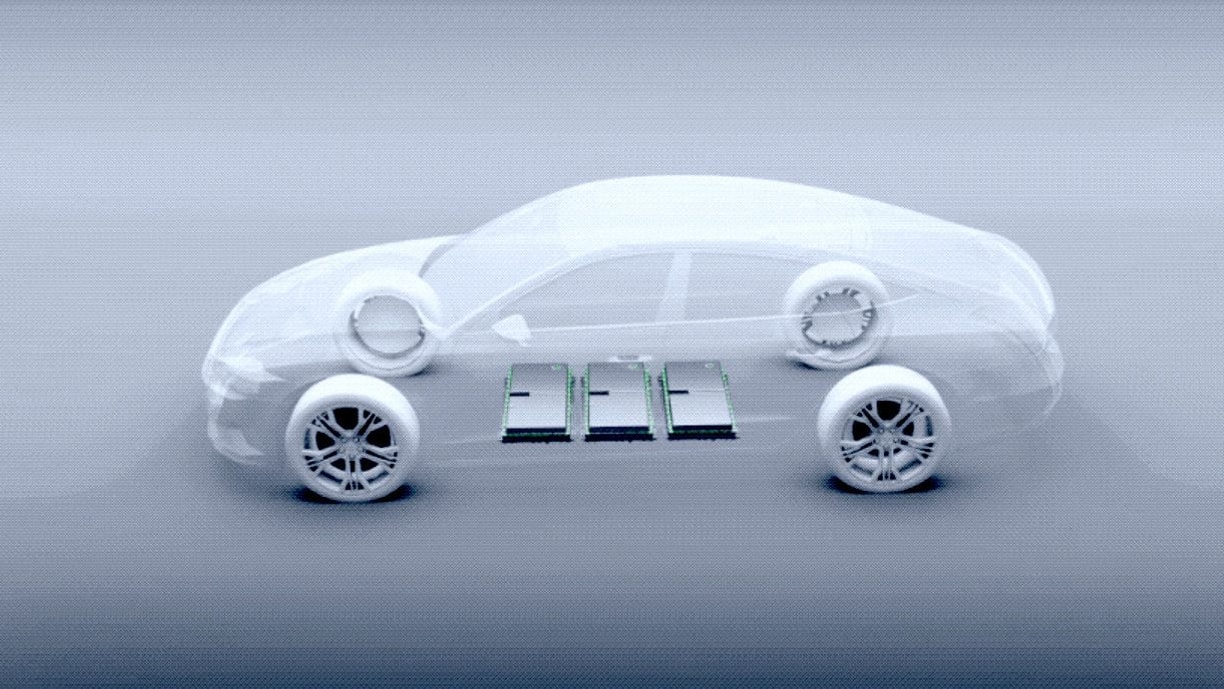
Manufacturing capacity for EV batteries is set to far outpace growth, noted BNEF last week—even including continued global EV sales growth.
- Never Miss a Green Car Reports Story I agree to receive emails from Green Car Reports. I understand that I can unsubscribe at any time. Privacy Policy. subscribe today

- 500e Reviews
2024 Fiat 500e First Drive Review: Reborn EV packs style, plays music out its bumper
You're not going to find a more charming electric city car than this one.

MIAMI – It's been hard times for Fiat fans. The brand discontinued its 500 model line after 2019, leaving only the four-door crossover-ized 500X on sale, and while it planned to introduce the replacement for its 500e electric city car around that time, a little pandemic threw a wrench in the works. Now, in 2024, the folks from Turin are finally ready to let Americans taste the Dolce Vita offered by that new 500e. Has the wait been worth it? I went to Miami to find out.
As you'd expect, the 2024 Fiat 500e is once again a fully electric city car. Unlike its predecessor (2013-2019), this 500e feels like a more complete EV package than a "compliance car" meant to satisfy regulations in states like California. The "New Fiat 500 " has been on sale in Europe since 2020, so while this is a new model for the United States, it's by now a well-established platform.
Like the old 500e, the 2024 500e features a front-wheel-drive layout, with a permanent magnet motor providing a whopping 117 horsepower and 162 pound-feet of torque. That motor is paired with a 42-kilowatt-hour battery (around 38 of which is usable, per Fiat engineers) for an EPA-estimated range of 141 with all-season tires . That doesn't sound like a lot – and it isn't – but this isn't a vehicle designed for long road trips or super-commuters. Fiat claims a 0-60 time of 8.5 seconds and a top speed of 94 mph, but I suspect that driving at that speed would be trouser-browningly sketchy.
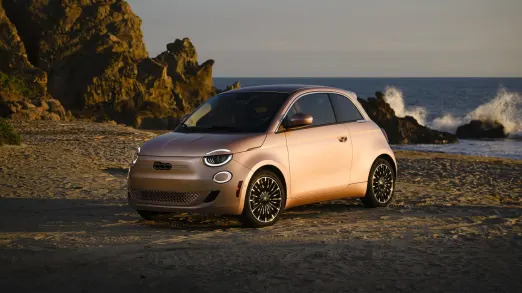
Not that the 500e feels unstable around town (Fiat didn't include any highway driving during my brief stint with the car). It offers the kind of quick, accurate steering and subcompact agility that made the original 500e so much more fun to drive than it needed to be. The steering is light but not overly so and offers a very tight turning circle of just 31.5 feet, making the "Oh wait, there's a parking spot! Get it!" maneuver much easier than it would be in a larger vehicle.
While the steering is awesome, the 500e's ride isn't as pleasant. It's not unladen-full-size-truck brutal by any means, but calling it busy over broken city streets feels like a charitable way of putting it. This isn't an expensive car with sophisticated suspension, and, again, given its intended use case, I wouldn't count the ride as a huge point against it.
One of the biggest leaps forward from the old 500e to this new version is the addition of DC fast charging capability. The 2024 model uses a CCS (combined charging system) plug for fast charging at up to 85 kilowatts. That sounds more like a drinking straw for electrons than the typical 150 or 350 kW firehose-like charging capabilities of most modern EVs, but it beats the Mini Cooper SE by 35 kW, and Fiat claims it'll charge from 10% to 80% in 35 minutes. Fiat is also leaning hard into Level 2 charging by giving 500e buyers a choice between a free Level 2 wall charger or $600 in charging credits with Stellantis’ partner Free2Move.
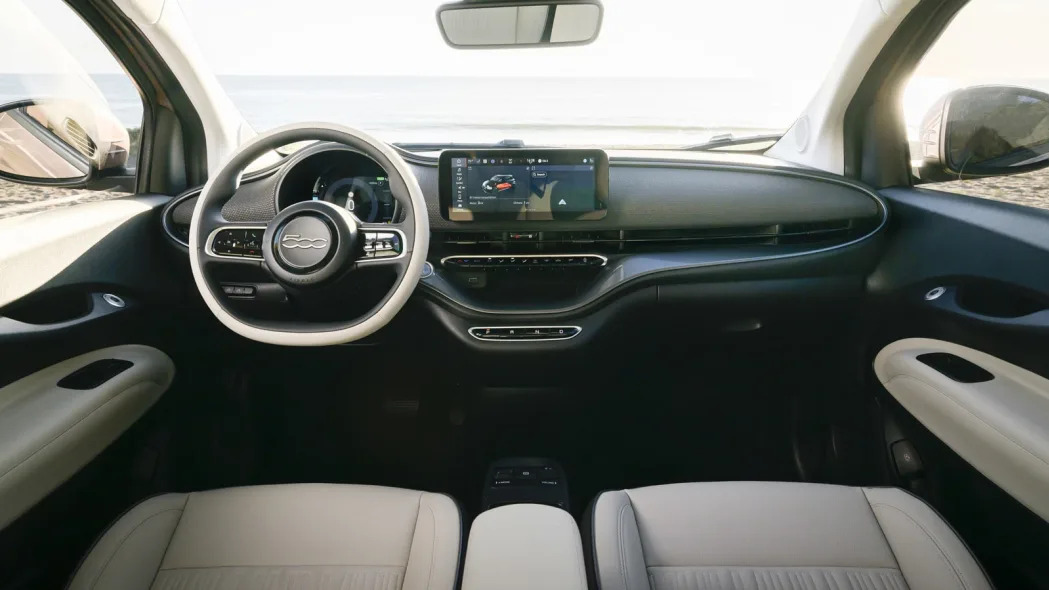
Being a simple car overall, the 500e offers three drive modes. There's Normal, Range and the questionably named Sherpa. Normal is fairly self-explanatory, Range chills out the acceleration for better efficiency, and Sherpa is like Range mode plus. It shuts down the HVAC system and gives you the least responsive accelerator pedal and maximum regenerative braking . Or in Fiat’s words, Sherpa mode takes “charge of the whole expedition and guides it to the destination” by adjusting those above parameters. Unlike EVs from other brands (looking at you, Germans) the 500e offers one-pedal driving, which is ideal for intra-city commuting.
The 500e has a skateboard-style chassis with the battery pack located under the floor of the passenger compartment. This is pretty standard stuff for nearly all built-to-purpose electric vehicles and offers the benefit of a very low center of gravity, which makes for a fun, agile driving experience. That’s good, because agility is what the 500e is all about.
The new 500e has grown in nearly every direction from its predecessor, but not by much. The difference is mostly felt inside, and that's a good thing. Up front, the new 500e offers up 39.3 inches of headroom (up 0.4 from the old 500e), 41.8 inches of legroom (plus 1.1 inch) and 49.8 and 51.1 inches of hip and shoulder room, respectively (plus 2 inches and 1.7 inches). That increase is welcome, especially as a very tall – 6 feet, 4 inches – individual, though as a passenger, I'd love it if its seat had the same height adjustment as the driver's seat. Back seat legroom is also up by 2 inches, but that still doesn’t make it anywhere you’d want people you like to spend an extended period of time. Cargo volume is predictably piccolo at just 7.5 cubic feet, up a half cube.
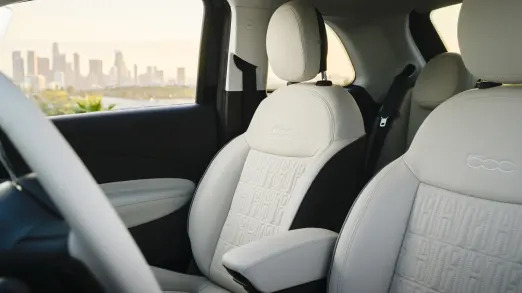
The 500e’s interior is basic with some unfortunately cheap-feeling materials, but it's aesthetically pleasing and not a horrible place to be. The seats are firm and lack real bolstering but are clad (in my base test vehicle) with cool-looking, embossed Fiat logo upholstery. There are plenty of places to store all your stuff, though only having one cupholder feels like a misstep for a vehicle in the U.S., no matter how small the car may be. The 500e also gets a wireless charging pad as standard.
From a tech standpoint, the 500e is similarly basic. The base RED model lacks features like adaptive cruise control or active lane keep assist, but still gets things like automatic emergency braking, blind-spot warning and lane departure alert. If you want more driver assistance features, jumping up to the "Inspired By" trims is the only way to get them. Infotainment is handled by Stellantis' typically excellent Uconnect 5 system, including a big-for-the-cabin 10.25-inch touchscreen I found to be crisp and responsive to touch inputs. Apple CarPlay and Android Auto are standard along with a perfectly serviceable stereo system; "Inspired By Music" models get a seven-speaker JBL system, but we're not driving those.
Something that Fiat is extremely proud of but that seemed absent on our test vehicles is the "Acoustic Vehicle Alert System," which plays a song on exterior speakers to warn pedestrians. This song, which you can listen to below, is called "The Sound of 500" and was written by Flavio Ibba-Marco Gualdi. It's supposed to play every time you set off until 12 mph or whenever you dip below that speed, then give way to a more typical white noise. Sadly, despite my best efforts, I was unable to trigger it. Perhaps it was a pre-production issue, but either way, the bumper concert will have to wait for another day.
One nice thing about the 500e is that the 2024 is slightly cheaper than the old model despite being better in nearly every measurable respect. It’s not cheap per se, given that Fiat thinks of itself as a premium brand in the United States. Still, with a sticker price of $34,095 (including a $1,595 destination fee) for the base (RED) version and $37,595 for the "Inspired By" versions, it's not completely egregious either. That's reinforced by the fact that the only options available on the RED or “Inspired by” trim levels are tire choice (summers or all-seasons) and which of the previously mentioned charging perks you want. Unfortunately, because the 500e is built in Italy (specifically, Fiat’s famous Mirafiori factory in Turin), it isn't eligible for the federal EV tax credit.
While not horrible, that price is more than you'd pay for the much quicker Mini Cooper SE and its 181 hp. You pay for that extra performance in a different way, though, with an EPA-estimated range of just 114 miles. The Mini starts at $31,895, but the base spec is somewhat barebones with 16-inch wheels and no Android Auto versus the Fiat's awesome-looking standard 17-inch units and wireless smartphone connectivity for both Apple and Android devices. The Mini also only fast charges at 50 kW, so if you plan to use public DC fast chargers, plan to spend more time waiting around in the Mini (and annoying everyone else waiting to charge).
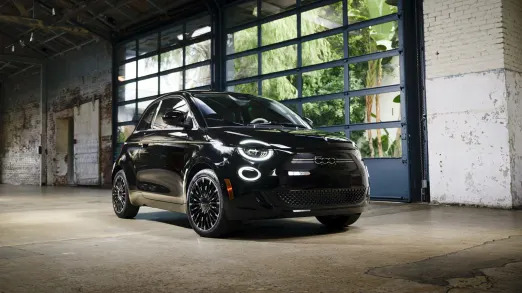
Fiat 500e Inspired by Music
Now, the answer to the great question: Was the 2024 Fiat 500e worth the four-year wait? In short, yeah. Fiat claims that it took the time between the initial announcement in 2020 and now to find and fix bugs with the vehicle and ensure it was as ready for the U.S. market as possible. In driving it, I found that, while still a little rough around the edges, it's an excellent choice for drivers fitting the 500e's narrow intended use case.
It's nimble, super-attractive (subjective, but important), offers a decent enough cabin and enough range and performance to not feel like a hindrance to traffic or a burden to your lifestyle. If you live in a densely populated urban area like New York or Los Angeles, the little Fiat will make life easier and more pleasant, but if you're somewhere with big distances between destinations, it's not the car for you.
I wouldn't call the refreshed 500e a home run or a shoo-in for huge sales, given its highly specific purpose, but I enjoyed my time with it and would recommend it to someone looking for a little bit of La Dolce Vita.
Featured Gallery Fiat 500e

FIAT 500e Information
Compare the 500e with similar vehicles.
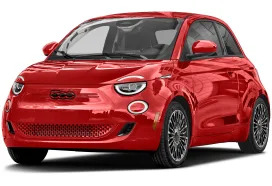
- All Model Years
- Rebates & Incentives
- News & Reviews
- Photos & Videos
- More 500e Information
- First Drives
- Economy Cars
Autoblog accepts vehicle loans from auto manufacturers with a tank of gas and sometimes insurance for the purpose of evaluation and editorial content. Like most of the auto news industry, we also sometimes accept travel, lodging and event access for vehicle drive and news coverage opportunities. Our opinions and criticism remain our own — we do not accept sponsored editorial.
- License License
- Facebook Share
- Twitter Share
- Tumblr Share
- Twitch Share
- Flipboard Share
- Instagram Share
- Newsletter Share
- Youtube Share
- Feeds Share

2024 FIAT 500e

Popular Vehicles
Popular new vehicles.
- 2023 Ford Bronco
- 2023 Toyota Camry
- 2023 Toyota Tacoma
- 2023 Ford F-150
- 2024 Toyota RAV4
- 2024 Ford Bronco
- 2023 Jeep Wrangler
- 2024 Toyota Camry
- 2024 Lexus GX 550
- 2023 Toyota 4Runner
Popular Used Vehicles
- 2022 Ford F-150
- 2021 Jeep Grand Cherokee
- 2022 Toyota 4Runner
- 2022 Honda Accord
- 2020 Honda Civic
- 2014 Chevrolet Silverado 1500
- 2014 Honda Civic
- 2018 Chevrolet Camaro
- 2014 Honda Accord
- 2021 Toyota Tundra
Popular Electric Vehicles
- 2023 Tesla Model 3
- 2017 Tesla Model S
- 2016 Tesla Model S
- 2024 Rivian R1T
- 2023 GMC HUMMER EV Pickup
- 2022 Tesla Model 3
- 2023 Rivian R1T
- 2020 Tesla Model 3
- 2024 GMC HUMMER EV Pickup
- 2023 Tesla Model S
Popular Truck Vehicles
- 2024 Chevrolet Silverado 1500
- 2024 Chevrolet Silverado 2500HD
- 2024 Toyota Tacoma
- 2024 Ford F-150
- 2023 Toyota Tundra
- 2023 Chevrolet Silverado 1500
Popular Crossover Vehicles
- 2023 Ford Bronco Sport
- 2024 Honda CR-V
- 2024 Hyundai Santa Fe
- 2024 Chevrolet Trax
- 2024 Honda Pilot
- 2023 Toyota RAV4
- 2024 Subaru Outback
- 2024 Kia Telluride
Popular Luxury Vehicles
- 2024 Porsche 911
- 2024 Lexus RX 350
- 2024 Land Rover Defender
- 2024 Land Rover Range Rover Sport
- 2014 Mercedes-Benz C-Class
- 2021 Lexus RX 350
- 2022 Lexus IS 350
- 2024 Mercedes-Benz GLC 300
- 2023 Porsche 911
Popular Hybrid Vehicles
- 2023 Ford Explorer
- 2024 Toyota Sienna
- 2022 Ford Explorer
- 2024 Toyota Tundra Hybrid
- 2024 Toyota RAV4 Hybrid
- 2023 Toyota Sienna
- 2024 Ford Explorer
Popular Makes
Featured makes, product guides.
- The Best Electric Bikes
- The Best Car Covers
- The Best Portable Air Compressors
- The Best Car GPS Trackers
Choose a Display Name
Please enter a display name

Sign in to post
Please sign in to leave a comment.
2025 Mercedes-Benz eSprinter First Drive: Electric Worker Bee Adds Features
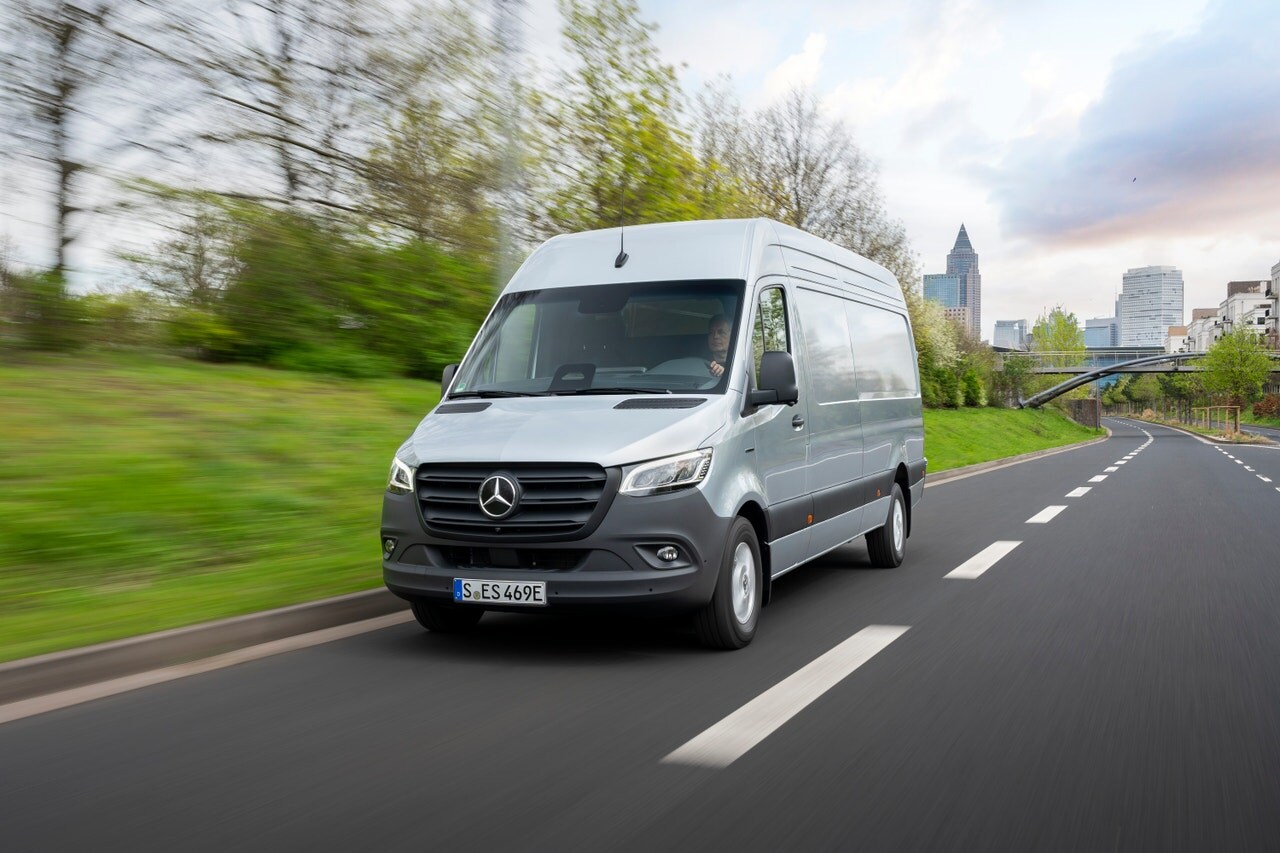
- More body style configurations are available on the 2025 eSprinter.
- The starting price is lower thanks to a new standard battery, but with less range.
- You'll find new driver assist features too.
The Mercedes-Benz eSprinter is essentially the large cargo/passenger van many know and love, but now with an electric powertrain. Mercedes introduced it for the 2024 model year and is now adding new versions to the eSprinter lineup for 2025. While the eSprinter costs significantly more than a comparable diesel-powered Sprinter up front, electrification comes with the long-term benefits of fuel savings and potentially simpler and less costly maintenance. That's enough to make it a compelling option for small-business owners and fleet operators.
Am I Ready for an EV?
- EV ownership works best if you can charge (240V) at home or at work This typically means a 240V home installation, but you could also have a similar setup at your office or other places your car is already parked for several hours each day. Don't expect a regular household outlet (120V) to suffice unless you've got a plug-in hybrid, in which case overnight charging at home is feasible.
- If you can’t charge at home, charging at a charging station could take at least 10x longer than at a gas station With public charging infrastructure still in its infancy, the user experience can be maddeningly inconsistent. Tesla owners tend to rave about the reliability and speed of the company's proprietary Supercharger stations, but rival DC fast options have thus far been plagued by technical issues and overcrowding. It's an evolving landscape and our best advice is to do your research on the available options for the EV you want to buy.
- Adding a 240V home charging system could cost up to $1,600 or more If your existing electrical service can handle the additional demands of EV charging, you may be able to add Level 2 charging at home for less than a grand, including installation. But your costs will multiply if you need to upgrade your electrical panel or add a dedicated circuit.

What's new for the 2025 eSprinter?
Previously, the eSprinter came exclusively in the 170-inch long-wheelbase, high-roof configuration fitted with a battery pack with a usable capacity of 113 kWh. That's still available for 2025. In addition, the 2025 eSprinter is now available with the van's shorter 144-inch wheelbase and standard-height roof. This version also comes with a smaller battery with a usable capacity of 81 kWh.
How much power and range does the eSprinter have?
The electric motor that drives the eSprinter's rear wheels produces 134 hp in standard output form, or up to 201 hp for the high-output motor. Torque for either motor peaks at 295 lb-ft, which is the important number for low-speed city street duty. That's pretty comparable to the power produced by the regular Sprinter's four-cylinder diesel engine, though the eSprinter is heavier because of the batteries.
There aren't any EPA range estimates available just yet, but we'd estimate somewhere around 120 miles for the small battery vans and up to 250 miles for vans with the large battery.
For charging, the eSprinter can accept Level 2 charging at up to 9.6 kW and comes standard with DC fast-charging capabilities at up to 50 kW. While this should suffice for most overnight charging scenarios, if you need to charge on the go quicker, you can upgrade to 115-kW DC fast charging. With the faster charging, Mercedes-Benz estimates a charge time of 42 minutes and 32 minutes, respectively, for the large and small battery to take you from 10% to 80% battery state of charge.

How does the eSprinter drive?
In general, it drives a lot like a regular Sprinter van fitted with the available high-output turbodiesel engine. The diesel has a torque advantage on paper but there is noticeable delay before it delivers full power. Torque from the eSprinter battery, however, is instant and allows for smoother acceleration, especially in stop-and-go conditions.
Braking is another differentiator. There are five settings of brake regeneration so the driver has options as to how much assistance the van offers. In its most aggressive level, D Auto, the vehicle will automatically brake based on traffic and road conditions. We liked using this mode when going downhill. But in traffic, the braking was unexpected and disconcerting.
There are also three drive mode options: Comfort, Eco and Max Range. Comfort offers full power. Eco mode reduces climate control use and softens the acceleration curve for the standard motor, while it limits drive power to 67% for the larger motor (about 134 hp or 100 kW). Max Range goes full turtle mode, dropping power to 80 kW for either motor (about 107 hp). It's slow, but it's efficient.

How's the interior?
Not a lot has changed on the interior for 2025 model year eSpinter. The cabin still is clearly one of a work van and, just as obviously, a Mercedes-Benz. Large areas of hard plastics cover the dash and door panels, all seemingly durable and easy to clean. Storage nooks, pockets and even a set of questionable cupholders by the front windshield reinforce its functional design.
Soft elbow pads on the armrests add a touch of luxury and comfort, as do the seats. And now you can opt for a heated seat for your front passenger, in case you aren't always driving solo. Primary controls for temperature and fan speeds are buttons and toggle switches built to a quality finish.
How's the eSprinter's tech?
There are more tech options available in 2025 that greatly enhance the safety and driving experience of eSprinter. You can now outfit eSprinter with a host of advanced driver aids including adaptive cruise control, lane keeping assistance and traffic sign recognition as well as a 360-degree surround-view camera, which significantly eases the stress of maneuvering through tight spots. We also love the digital rearview mirror, particularly for vehicles of this type that would otherwise have to rely solely on sideview mirrors to see what's behind you.
Mercedes-Benz's excellent MBUX infotainment system remains an option in eSprinter, and it's been improved for 2025. The 10.25-inch wide display remains in its prominent place on the van's dash and can be managed with touch controls on the new multi-function steering wheel or by voice control. The system features faster computing power and improved menu navigation now certain voice-controlled functions no longer require the use of a "Hey Mercedes" wake word prompt. The MBUX system serves as a the hub for Mercedes me connect, a subscription service offering features like remote access to charging data, climate control preconditioning, navigation route adjustments based on current battery range, and a prepaid charge card for in-network EV chargers.

What about payload and towing capacities?
If you're looking to maximize payload capacity, the eSprinter with the smaller battery and the shorter roof and wheelbase accommodates up to 3,516 pounds. If you need more space but don't need maximum range, then the tall-roof, long-wheelbase eSprinter with the small battery might suit you with up to 3,120 pounds of payload capacity. If you need the range to live out your best "van life," the larger battery will drop your payload limits to 2,624 pounds because, hey, batteries are heavy. Cargo space ranges from as little as 319 cubic feet to as much as 488 cubic feet. Maximum tow capacity for the eSprinter ranges from 5,000 pounds (small battery), which now matches the diesel Sprinter, to 4,277 pounds (large battery).
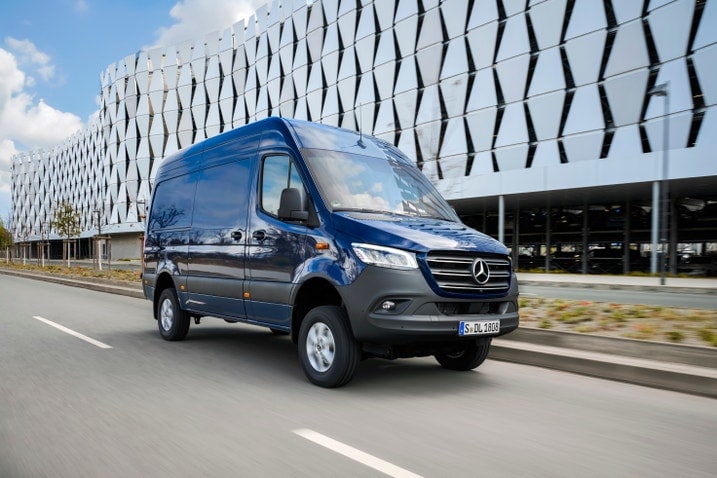
Edmunds says
The new configurations and options for the 2025 eSprinter make it more suitable to a wider range of customers. It's still slightly less capable than a regular Sprinter for maximum hauling, but we think electrification improves the driving experience overall and we would prefer it if we were primarily operating within city limits.
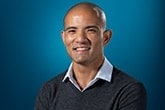
Jonathan Elfalan has worked in the automotive industry since 2005. As a director of vehicle testing at Edmunds, Jonathan has tested and reviewed thousands of cars and written thousands of car-related articles over the course of his career. Jonathan got his start testing cars for Road & Track magazine as a newly minted mechanical engineer grad from University of California, Irvine, and has also contributed to Motor Trend and the Associated Press. He likes to say he learned to drive a manual transmission in a rear-wheel-drive mid-engine vehicle but often omits it was his family's 1991 Toyota Previa minivan.
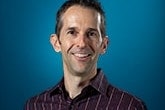
Brent Romans has worked in the automotive industry since 1996. He has written or edited thousands of expert car reviews and road-tested hundreds of vehicles over the course of his career. Brent is a senior manager of written content at Edmunds and previously contributed to publications such as Super Street and Petersen's 4-Wheel & Off-Road. His personal car is a 2019 BMW M2 Competition, which he bought for its ideal combination of performance, style and practicality.
Related information
Other models.
- Used Hyundai Elantra-Gt in The Villages, FL
- Used Hyundai Tucson-Plug-In-Hybrid in Norwalk, CT
- Used Toyota Fj-Cruiser in Culver City, CA
- New BMW Alpina-B8-Gran-Coupe for Sale in Columbia, TN
- New Ford Bronco for Sale in Portland, OR
- Used Ford F-250 in Bellmore, NY
- Used Infiniti M56 in Ormond Beach, FL
- Used Mercedes-Benz G-Class in San Gabriel, CA
- New Nissan Rogue for Sale in Santa Fe, NM
- Used Toyota Mr2-Spyder in Mayfield, KY
Recent automotive news
- 2024 Mazda CX-90 Plug-in Hybrid Joins Our Fleet for a Year
- Nissan and Mitsubishi Want to Bring an Electric Truck to America
- Rolls-Royce Spectre Is Quick, Quiet and Beat Its EPA Range in Our Testing
- 2024 Fiat 500e First Drive: Little EV, Big Personality
- 2025 Toyota 4Runner First Look: Toyota's Bronco-Fighter Is Reborn
- 2025 Buick Enclave First Look: Hands-Free Driving and a Swanky Interior
- Wildcat Concept Will Continue to Influence Future Buick Designs
- 2025 Acura MDX Brings an Edgier Look and Updated Technology
- 2024 Jeep Gladiator NightHawk Gets Dark Just in Time for the Eclipse
- Corvette E-Ray vs. McLaren 750S: One Of the Quickest U-Drags Ever
Popular new car reviews and ratings
- 2025 Audi Q5 News
- 2023 Honda Civic
- Audi Q5 2023
- Kia Rio 2023
- BMW M3 2023
- Car A5 Audi
- Hyundai Elantra 2023
- 2025 Toyota Tundra News
- 2023 Mazda Protege
- Nissan GT-R 2024
Lease deals by make
- Cadillac Lease Deals
- Audi Lease Deals
- Buick Lease Deals
- Honda Lease Deals
- Chevrolet Lease Deals
- BMW Lease Deals
- Ford Lease Deals
- GMC Lease Deals
- Hyundai Lease Deals
- Genesis Lease Deals
Lease deals by model
- Dodge Charger Lease Deals
- Chevrolet Equinox Lease Deals
- Chevrolet Silverado Lease Deals
- Ford F-150 Lease Deals
- Ford Explorer Lease Deals
- Ford Escape Lease Deals
- Chevrolet Tahoe Lease Deals
- Chevrolet Traverse Lease Deals
- Dodge Challenger Lease Deals
- Ford Mustang Lease Deals
Join Edmunds
Receive pricing updates, shopping tips & more!
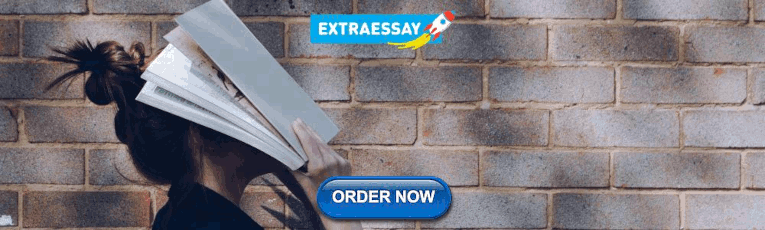
IMAGES
VIDEO
COMMENTS
Multiple literature reviews have integrated sustainability performances into business in different fields ( Morioka and de Carvalho, 2016; Siegel et al., 2019 ); however, such reviews are missing in the electric field domain. Only a few literature reviews identified on the electric vehicles covering limited aspects of adoption.
Electric Vehicles (EVs) are gaining momentum due to several factors, including the price reduction as well as the climate and environmental awareness. This paper reviews the advances of EVs regarding battery technology trends, charging methods, as well as new research challenges and open opportunities. More specifically, an analysis of the worldwide market situation of EVs and their future ...
First introduced at the end of the 1800s, electric vehicles (EVs) 12 have been experiencing a rise in popularity over the past few years as the technology has matured and costs (especially of batteries) have declined substantially. Worldwide support for clean transportation options (i.e. low emissions of greenhouse gasses [GHG] to mitigate climate change and criteria pollutants) has promoted ...
This paper reviews the technical background of electric vehicle technology and its applications. A number of important concepts frequently used in this field are explained, and the technical details, including the theoretical principles, are given alongside practical systems pertaining to several kinds of electric charging piles. It critically appraises a number of state-of-the-art research ...
1. Introduction. Many governments have initiated and implemented policies to stimulate and encourage electric vehicle (EV) production and adoption (Sierzchula, Bakker, Maat, & Van Wee, 2014 ). The expectation is that better knowledge of consumer preferences for EV can make these policies more effective and efficient.
Electric vehicle (EV) disposition may challenge serious environmental issues such as excessive dependence on oil, especially in the transport sector. Despite this understanding, the adoption intention has been disappointing to date. This review tries to present a comprehensive overview of the methodologies, theories, and variables used in 57 peer-reviewed articles published between 2015 and ...
This study addresses the pressing need to evaluate the life cycle assessment (LCA) of electric vehicles (EVs) in comparison to traditional vehicles, amid growing environmental concerns and the quest for sustainable transportation alternatives. Through a systematic four-stage literature review, it strives to provide essential insights into the environmental impact, energy consumption, and ...
Abstract. Scholarly research on the topic of electric vehicles has witnessed a dramatic increase in the current decade; however, reviews that synthesize and integrate these findings comprehensively have been lacking. This study is an attempt at filling in that void through an integrative review methodology. It includes an integrative review of ...
This literature review examines consumer behaviour in the electric car market using the techniques of bibliometric and thematic analysis. It offers guidance for future researchers regarding consumer characteristics, theories, context, and methodologies.
Electric vehicles: Literature review of technology costs and carbon emissions Authors: Paul Wolfram and Nic Lutsey Date: 15 July 2016 Keywords: Electric vehicles, well-to-wheel greenhouse gas emissions, technology costs, lithium-ion battery, fuel cell, plug-in hybrid, renewable electricity 1. in a lower-carbon 2020-2030 new SUMMARY
1. SUMMARY The European new vehicle CO2 regulat ion (with a mandatory target value of 95 grams of CO2 per kilometer by 2021 for passenger cars) is currently in the process of being extended to 2025. In this context, one of the key questions is at what point a significant uptake of the electric vehicle market is to be expected. In order to help inform this debate about how electric vehicle ...
In this context, this paper aims to evaluate the factors that impact electric vehicles (EV) adoption. A systematic review was conducted considering peer-reviewed papers published in English from 2016 to 2020, and results were separated into clusters to enable the assessment.
This review tries to present a comprehensive overview of the methodologies, theories, and variables used in 57 peer-reviewed articles published between 2015 and 2022 covering the main forms of consumer adoption of EVs, consisting of purchase as well as behavioral and usage intentions.
A Literature Review on Electric Vehicles: Architecture, Electrical Machines for Power Train, Converter Topologies and Control Techniques Abstract: Electric Vehicles are becoming order of the day due to its various advantages over normally used IC engine vehicle. The bloom of this particular field is making environment more green and causes less ...
Consumer Adoption of Electric V ehicles: A Systematic. Literature Review. Paweł Bryła 1, * , Shuvam Chatterjee 2 and Beata Ciabiada-Bryła 3. 1 Department of International Marketing and ...
The study has different significant implications for the literature on electric vehicles' (EVs) energy efficiency and emission reduction effect. It will contribute to the existing literature and research in different ways. ... A bibliometric review on electric vehicle (EV) energy efficiency and emission effect research. Environ Sci Pollut Res ...
Electric Vehicles in India: A Literature Review. March 2021. Conference: 7th International Conference on "New Frontier in Energy, Engineering and Science (NFEES), 19-20 March 2021. At: Jaipur ...
Integration of electric vehicles (EVs) into the smart grid has attracted considerable interest from researchers, governments, and private companies alike. Such integration may bring problems if not conducted well, but EVs can be also used by utilities and other industry stakeholders to enable the smart grid. This paper presents a systematic literature review of the topic and offer a research ...
An IBM consumer survey (Gyimesi & Viswanathan, 2011) similarly found that 45% of the surveyed drivers had little to no understanding of electric vehicles. Federal incentives in the United States amount to up to $7,500 per electric vehicle, and additional state incentives typically amount to more than $2,000.
This paper reviews the current literature on plug-in electric vehicles (PEVs) with a focus on issues and solutions related to vehicle deployment and integration with the U.S. electrical grid. It is a companion to the Center's "Plug-in Electric Vehicles Market: State of Play." The subjects covered include vehicles, electricity, the passenger vehicle market, and public […]
There exists a rich body of literature on the adoption of electric vehicles. However, most of these studies explicitly focus on the adoption patterns of electric four-wheelers (Bhat and Verma 2022; Coffman et al. 2017; Liao et al. 2017; Rezvani et al. 2015; Singh et al. 2020a).Despite the importance that the electrification of two-wheeler mobility ought to be given, the factors influencing the ...
Switching to EVs is an important part of fighting climate change, but far from the only change that needs to happen. The digital story was edited by Malaka Gharib. The visual editor is Beck Harlan ...
The state of the Model 3 vs Model 2: A new Rear Wheel Drive Model 3 in inventory starts at about $35,000. With a $7,500 incentive distributed over the term of a 36-month lease and 10,000 miles per ...
This study reviews the extant literature in the field of electric vehicle (EV) adoption that has employed statistical approaches to analyse actual market data. ... Consumer preferences for electric vehicles: a literature review. Transport Reviews, 37 (3) (2017), pp. 252-275, 10.1080/01441647.2016.1230794. View article View in Scopus Google ...
The 2024 Fiat 500e is also much, much better than the first-generation 500e EV sold between 2013 and 2019. Converted from the gas-fed 500, that one had just 84 to 87 miles of range and was created ...
By Mark Vaughn Published: Apr 17, 2024. VIEW PHOTOS. Lucid. Lucid wants to sell more of its Air sedans, so it's offering a sub-$70k model, a $649-a-month lease, and as much performance as you can ...
67. photos. The good folks at Hyundai have scrambled my brain with the 2025 Ioniq 5 N, a devilish little electric compact crossover that might just be a motorsports game-changer. Most electric ...
I went to Miami to find out. As you'd expect, the 2024 Fiat 500e is once again a fully electric city car. Unlike its predecessor (2013-2019), this 500e feels like a more complete EV package than a ...
4/17/2024. More body style configurations are available on the 2025 eSprinter. The starting price is lower thanks to a new standard battery, but with less range. You'll find new driver assist ...
Key insight 1: Electric mobility challenges current business models, while making it necessary for companies to capitalize on new sources of value. Electric mobility, in particular electric vehicles as part of it, induce a complex system of interdependent actors and elements.