
Want to create or adapt books like this? Learn more about how Pressbooks supports open publishing practices.
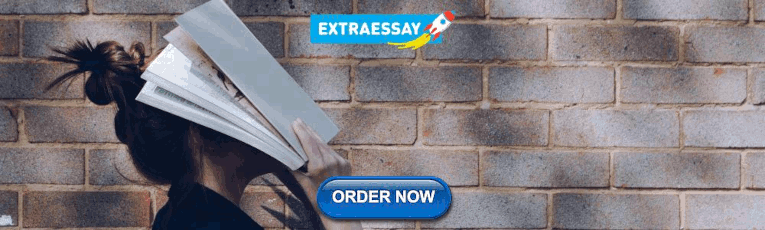
7.5 Testing the Red Queen Hypothesis
The Red Queen hypothesis—that sex evolved to combat our coevolving pathogens—can be tested by analyzing a few key predictions of this hypothesis:
- Sex is most beneficial where there is a high risk of infection
- Pathogens are more likely to attack common phenotypes (for example, clones) in a population, as opposed to the less-common counterparts (such as those that resulted from sex)
- In sexually reproducing populations, individuals choose mates that maximize diversity in their offspring
Note that all of these predictions implicitly rely on the heritability of being healthy (in this case, the ability to combat pathogens); specifically, parents must be able to pass along to their offspring genes for avoiding pathogens. Testing these predictions has resulted in several lines of evidence supporting the Red Queen hypothesis.
Prediction 1: Sex is most beneficial where there is a high risk of infection
An excellent system for testing this prediction involves a flatworm parasite in the genus Microphallus, a duck, and a small mud snail ( Potamopyrgus antipodarum ; Figure 7.7). This species of snail is able to reproduce sexually or asexually. The extent of sexual reproduction in a population of snails can be quantified by counting the number of males—asexual snails are all female.
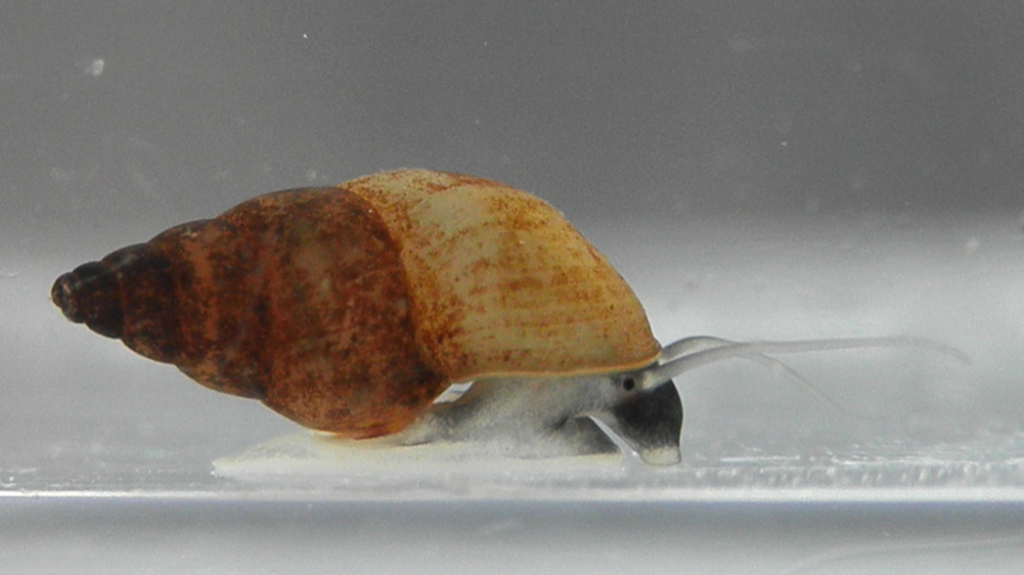
The flatworm’s life cycle begins inside of the snail, where the worm emerges from its egg. Infected snails are consumed by ducks. Once in the duck’s intestine, adult worms have sex and produce eggs. Flatworm eggs are released, with duck feces, into the water, where they are ingested by snails and the cycle continues (Figure 7.8). Snails are harmed by this flatworm, largely because a symptom of infection is sterilization (the flatworm’s scientific name, Microphallus , translates to “small penis”).
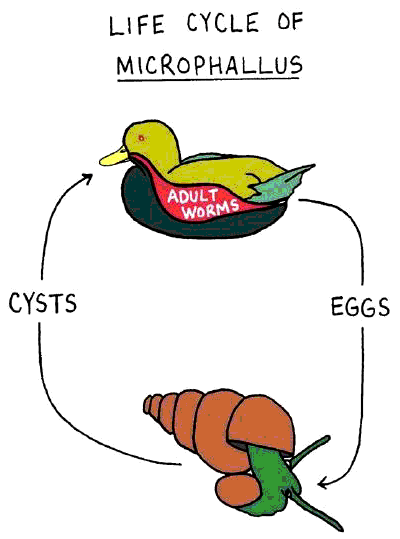
Observations of this system in two New Zealand lakes (Alexandrina and Kaniere) revealed that snails are more likely to be sexual (measured by frequency of males) in shallow waters, where ducks feed, than in deeper waters, where ducks do not feed (Figure 7.9).
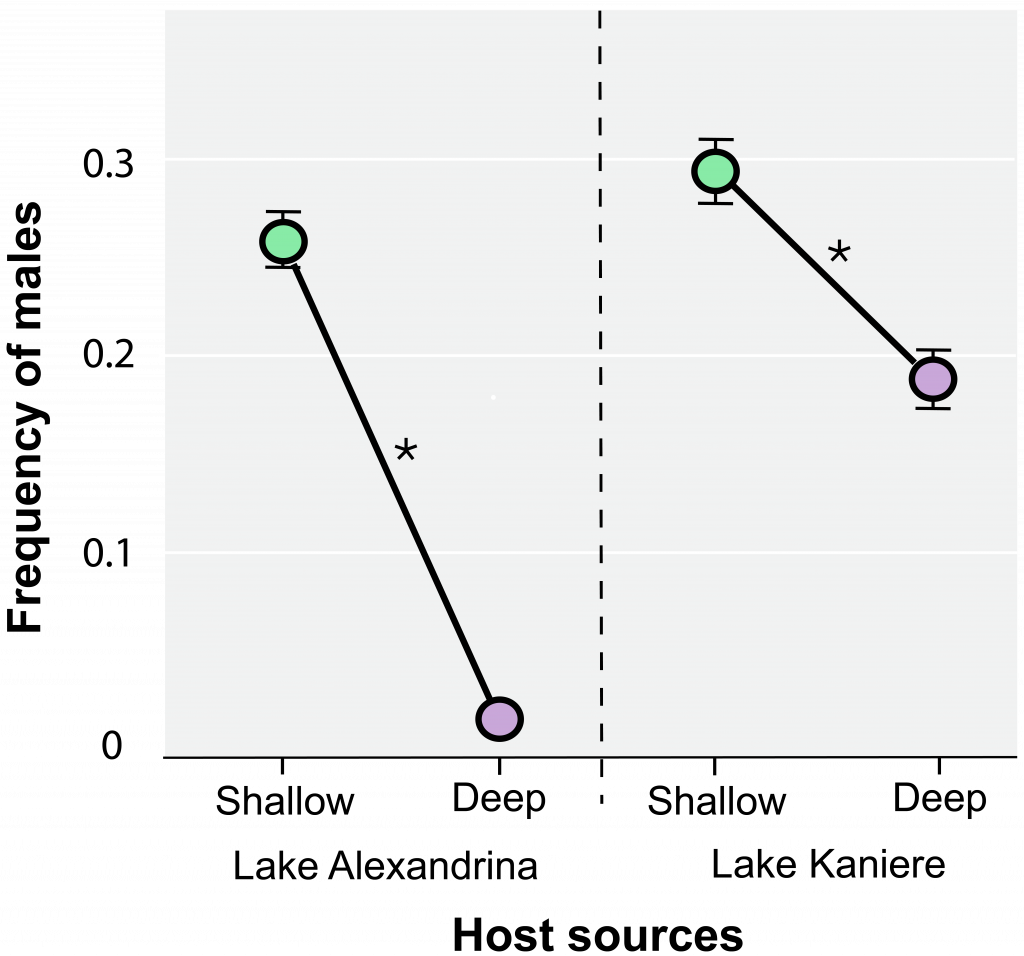
These results suggest that coevolutionary pressure is greater on the snails in the shallows, presumably because the feeding ducks effectively “close the circle” on the worm’s life cycle. Finally, higher infection rates in the shallows indicate that, in support of Prediction 1, above, sex is most beneficial where there is a high risk of infection.
Prediction 2: Pathogens are more likely to attack common phenotypes in a population, as opposed to the less-common counterparts
In the Mexican desert there are isolated pools inhabited by a species of minnow. Within these pools, populations of asexually reproducing individuals exist alongside sexually reproducing individuals. Fish in these ponds exhibit “black spot disease,” which is caused by a parasitic flatworm. Investigators have observed the frequency of sexual and asexual fish and the number of black spots in each type of fish in these ponds. Clonal fish are likely to have the most common phenotype in these ponds (as they are genetically identical to each other), while the sexually reproducing fish will have a wide variety of infrequent phenotypes. As the Red Queen predicts, the common type of fish (usually one of the clonal species) had the highest number of parasitic spots. In ponds where there was a genetically diverse, sexually reproducing population, the sexual fish had fewer spots.
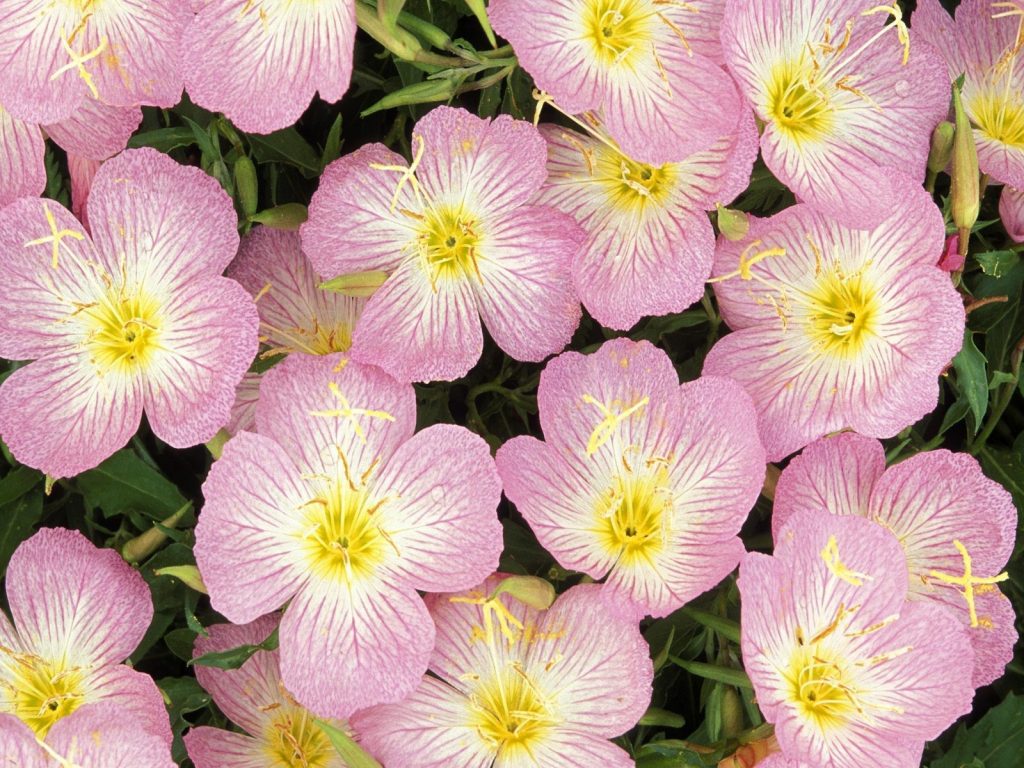
Additional evidence comes from the evening primrose (figure 7.10), a flowering plant that–like the minnows, snails, and water fleas discussed above–exists in sexual and asexual forms. Evening primrose can be damaged by mildew from a pathogenic fungus. The plants produce an enzyme protein called chitinase to defend themselves against this fungus. A recent comparison indicated that the sexually reproducing primrose plants had greater variety in the gene that codes for chitinase than did the asexual plants. In addition, the overall amount of chitinase expressed was higher in the sexual plants than in the asexuals. Finally, the researchers found that the plants that were more resistant to mildew damage had higher fitness (they produced more fruit, and thus more offspring) in the presence of that pathogen. In evening primrose, greater diversity in a key gene renders an individual less susceptible to a pathogen, supporting the prediction that parasites are more likely to attack the most common phenotype in a population, and providing additional evidence for The Red Queen.
Know Your Pathogens
A pathogen is something that infects and causes a fitness cost in another organism. Pathogens come in a wide variety; some of them are not even considered living!
Prions – Prions are non-living infectious agents that are misfolded proteins.
Viruses – Whether you consider viruses alive or not depends on your definition of life. Viruses are protein-encased DNA or RNA entities that hijack a cell’s replication machinery to reproduce. Viral infections include influenza, HIV, HPV, and herpes.
Fungal pathogens – Fungi are responsible for a variety of infections including mildew, thrush, athlete’s foot and smut.
Bacteria – Bacteria are prokaryotic organisms that occur everywhere. There are more bacteria in and on you than there are cells in your body. Fortunately, the vast majority of bacteria are benign. However, some bacteria cause problems such as urinary-tract infections, some kinds of pneumonia, ear infections, pertussis (whooping cough), chlamydia, gonorrhea, and syphilis.
Protists – Protists are single-celled eukaryotes that cause diseases such as malaria and amoebic dysentery.
Animals –Common animal pathogens include lice, many types of worms, and parasitic wasps.
Prediction 3: In sexually reproducing populations, individuals choose mates that maximize diversity in their offspring
If there is a fitness advantage to diversity, parents can best maximize their offspring’s potential (and have more grand-offspring) with careful mate choice. There are numerous examples of organisms preferring mates that increase offspring diversity, and shunning mates that might do the opposite. Even many hermaphrodites, with both male and female sex organs, seek other hermaphrodites for copulation…even if they are capable of self-fertilization.
An excellent model for studying mate choice is Atlantic Salmon, an important commercial fish that lives its life in the ocean and returns to freshwaters to mate (or spawn ). Sofia Consuegra and Carlos Garcia de Leaniz compared offspring diversity of salmon that were mated in a commercial fish hatchery (and unable to choose their mates) against that of salmon allowed to choose mates in the wild. The hatchery-spawned fish exhibited lower diversity than did the wild-spawned fish. Furthermore, hatchery-spawned fish displayed a greater number of roundworm parasites ( Anisakis ) then did their wild-spawned counterparts (figure 7.11). These results support the prediction that individuals will choose mates that maximize diversity in their offspring. Also, this work adds fuel to The Red Queen hypothesis by illustrating a potential benefit to Atlantic Salmon–namely, parasite avoidance.
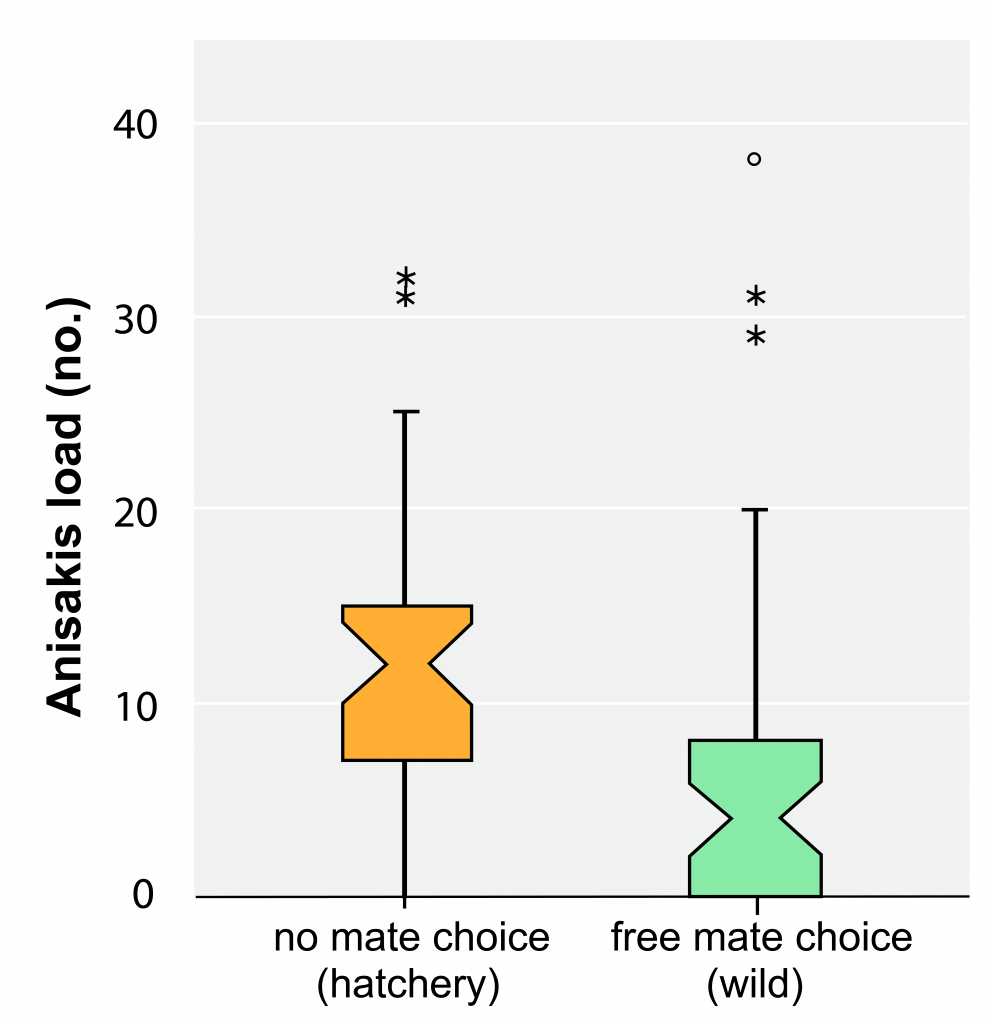
Check Yourself
- By Michal Maňas - Maňas M. (2014). "Photo of the day (35): Potamopyrgus antipodarum". Blog about gastropods. http://gastropods.wordpress.com https://gastropods.files.wordpress.com/2014/10/potamopyrgus-antipodarum.png, CC BY 4.0, https://commons.wikimedia.org/w/index.php?curid=36715581 ↵
The Evolution and Biology of Sex Copyright © 2020 by Sehoya Cotner and Deena Wassenberg is licensed under a Creative Commons Attribution-NonCommercial 4.0 International License , except where otherwise noted.
What Is the Red Queen Hypothesis?
Anup Shah/Getty Images
- Natural Selection
- History Of Life On Earth
- Human Evolution
- Evolution Scientists
- The Evidence For Evolution
- Habitat Profiles
- Marine Life
- M.A., Technological Teaching and Learning, Ashford University
- B.A., Biochemistry and Molecular Biology, Cornell University
Evolution is the changing in species over time. However, with the way ecosystems work on Earth, many species have a close and important relationship with each other to ensure their survival. These symbiotic relationships, such as the predator-prey relationship, keep the biosphere running correctly and keep species from going extinct. This means as one species evolves, it will affect the other species in some way. This coevolution of the species is like an evolutionary arms race that insists that the other species in the relationship must also evolve to survive.
The “Red Queen” hypothesis in evolution is related to the coevolution of species. It states that species must continuously adapt and evolve to pass on genes to the next generation and also to keep from going extinct when other species within a symbiotic relationship are evolving. First proposed in 1973 by Leigh Van Valen, this part of the hypothesis is especially important in a predator-prey relationship or a parasitic relationship.
Predator and Prey
Food sources are arguably one of the most important types of relationships in regards to survival of a species. For instance, if a prey species evolves to become faster over a period of time, the predator needs to adapt and evolve to keep using the prey as a reliable food source. Otherwise, the now faster prey will escape, and the predator will lose a food source and potentially go extinct. However, if the predator becomes faster itself, or evolves in another way like becoming stealthier or a better hunter, then the relationship can continue, and the predators will survive. According to the Red Queen hypothesis, this back and forth coevolution of the species is a constant change with smaller adaptations accumulating over long periods of time.
Sexual Selection
Another part of the Red Queen hypothesis has to do with sexual selection. It relates to the first part of the hypothesis as a mechanism to speed up evolution with the desirable traits. Species that are capable of choosing a mate rather than undergoing asexual reproduction or not having the ability to select a partner can identify characteristics in that partner that are desirable and will produce the more fit offspring for the environment. Hopefully, this mixing of desirable traits will lead to the offspring being chosen through natural selection and the species will continue. This is a particularly helpful mechanism for one species in a symbiotic relationship if the other species cannot undergo sexual selection.
Host and Parasite
An example of this type of interaction would be a host and parasite relationship. Individuals wanting to mate in an area with an abundance of parasitic relationships may be on the lookout for a mate that seems to be immune to the parasite. Since most parasites are asexual or not able to undergo sexual selection, then the species that can choose an immune mate has an evolutionary advantage. The goal would be to produce offspring that have the trait that makes them immune to the parasite. This would make the offspring more fit for the environment and more likely to live long enough to reproduce themselves and pass down the genes.
This hypothesis does not mean that the parasite in this example would not be able to coevolve. There are more ways to accumulate adaptations than just sexual selection of partners. DNA mutations can also produce a change in the gene pool only by chance. All organisms regardless of their reproduction style can have mutations happen at any time. This allows all species, even parasites, to coevolve as the other species in their symbiotic relationships also evolve.
- What Is the Evolutionary Arms Race?
- What Is Coevolution? Definition and Examples
- Symbiogenesis
- External Hierarchy of Life
- An Introduction to Evolution
- Parasitism: Definition and Examples
- Mutualism: Symbiotic Relationships
- Descent with Modification
- How Artificial Selection Works With Animals
- Tips on Winning the Debate on Evolution
- The 5 Types of Selection
- Survival of the Fittest vs. Natural Selection
- 6 Things You Should Know About Biological Evolution
- Jellyfish Facts: Habitat, Behavior, Diet
- Bacteria: Friend or Foe?
- Chromosome Mutations

Want to create or adapt books like this? Learn more about how Pressbooks supports open publishing practices.
41 Testing the Red Queen Hypothesis
The Red Queen hypothesis—that sex evolved to combat our coevolving pathogens—can be tested by analyzing a few key predictions of this hypothesis:
- Sex is most beneficial where there is a high risk of infection
- Pathogens are more likely to attack common phenotypes (for example, clones) in a population, as opposed to the less-common counterparts (such as those that resulted from sex)
- In sexually reproducing populations, individuals choose mates that maximize diversity in their offspring
Note that all of these predictions implicitly rely on the heritability of being healthy (in this case, the ability to combat pathogens); specifically, parents must be able to pass along to their offspring genes for avoiding pathogens. Testing these predictions has resulted in several lines of evidence supporting the Red Queen hypothesis.
Prediction 1: Sex is most beneficial where there is a high risk of infection
An excellent system for testing this prediction involves a flatworm parasite in the genus Microphallus, a duck, and a small mud snail ( Potamopyrgus antipodarum ; Figure 7.6). This species of snail is able to reproduce sexually or asexually. The extent of sexual reproduction in a population of snails can be quantified by counting the number of males—asexual snails are all female.
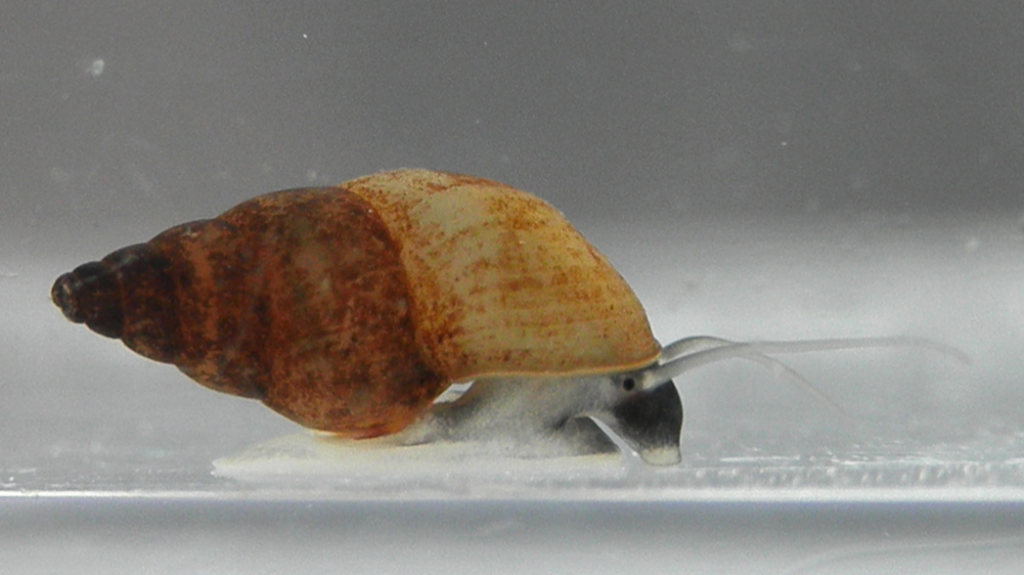
The flatworm’s life cycle begins inside of the snail, where the worm emerges from its egg. Infected snails are consumed by ducks. Once in the duck’s intestine, adult worms have sex and produce eggs. Flatworm eggs are released, with duck feces, into the water, where they are ingested by snails and the cycle continues (Figure 2). Snails are harmed by this flatworm, largely because a symptom of infection is sterilization (the flatworm’s scientific name, Microphallus , translates to “small penis”).
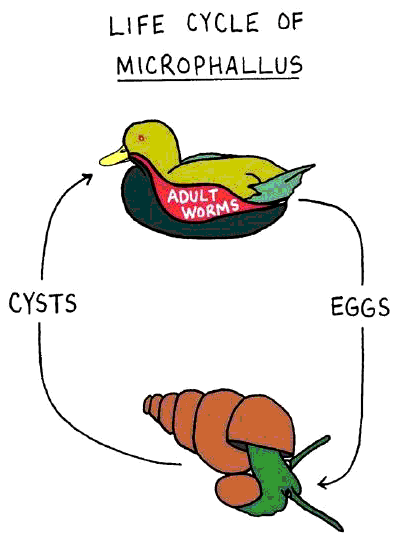
Observations of this system in two New Zealand lakes (Alexandrina and Kaniere) revealed that snails are more likely to be sexual (measured by frequency of males) in shallow waters, where ducks feed, than in deeper waters, where ducks do not feed (Figure 3).
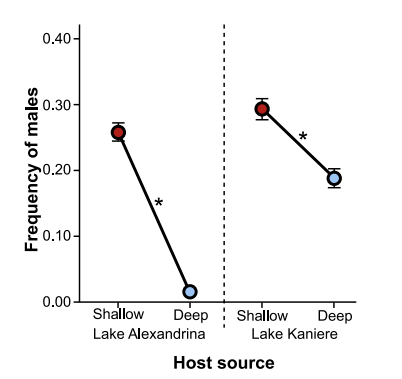
These results suggest that coevolutionary pressure is greater on the snails in the shallows, presumably because the feeding ducks effectively “close the circle” on the worm’s life cycle. Finally, higher infection rates in the shallows indicate that, in support of Prediction 1, above, sex is most beneficial where there is a high risk of infection.
Prediction 2: Pathogens are more likely to attack common phenotypes in a population, as opposed to the less-common counterparts
In the Mexican desert there are isolated pools inhabited by a species of minnow. Within these pools, populations of asexually reproducing individuals exist alongside sexually reproducing individuals. Fish in these ponds exhibit “black spot disease,” which is caused by a parasitic flatworm. Investigators have observed the frequency of sexual and asexual fish and the number of black spots in each type of fish in these ponds. Clonal fish are likely to have the most common phenotype in these ponds (as they are genetically identical to each other), while the sexually reproducing fish will have a wide variety of infrequent phenotypes. As the Red Queen predicts, the common type of fish (usually one of the clonal species) had the highest number of parasitic spots. In ponds where there was a genetically diverse, sexually reproducing population, the sexual fish had fewer spots.
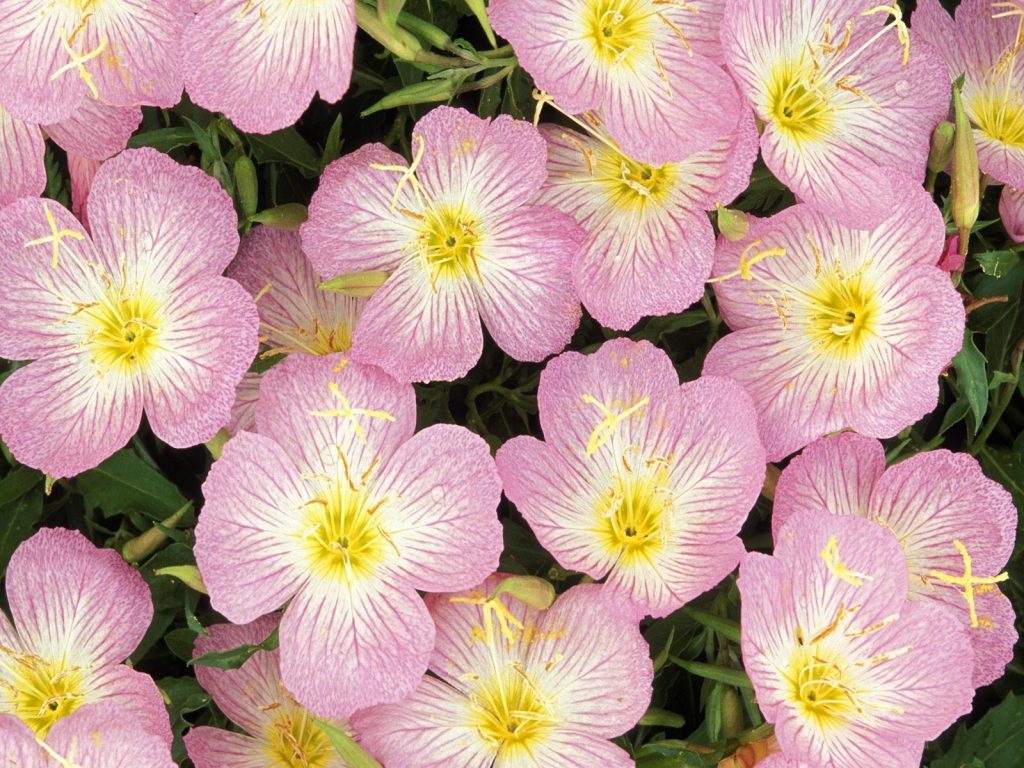
Additional evidence comes from the evening primrose (Figure 4), a flowering plant that–like the minnows, snails, and water fleas discussed above–exists in sexual and asexual forms. Evening primrose can be damaged by mildew from a pathogenic fungus. The plants produce an enzyme protein called chitinase to defend themselves against this fungus. A recent comparison indicated that the sexually reproducing primrose plants had greater variety in the gene that codes for chitinase than did the asexual plants. In addition, the overall amount of chitinase expressed was higher in the sexual plants than in the asexuals. Finally, the researchers found that the plants that were more resistant to mildew damage had higher fitness (they produced more fruit, and thus more offspring) in the presence of that pathogen. In evening primrose, greater diversity in a key gene renders an individual less susceptible to a pathogen, supporting the prediction that parasites are more likely to attack the most common phenotype in a population, and providing additional evidence for The Red Queen.
Know Your Pathogens
A pathogen is something that infects and causes a fitness cost in another organism. Pathogens come in a wide variety; some of them are not even considered living!
Prions – Prions are non-living infectious agents that are misfolded proteins.
Viruses – Whether you consider viruses alive or not depends on your definition of life. Viruses are protein-encased DNA or RNA entities that hijack a cell’s replication machinery to reproduce. Viral infections include influenza, HIV, HPV, and herpes.
Fungal pathogens – Fungi are responsible for a variety of infections including mildew, thrush, athlete’s foot and smut.
Bacteria – Bacteria are prokaryotic organisms that occur everywhere. There are more bacteria in and on you than there are cells in your body. Fortunately, the vast majority of bacteria are benign. However, some bacteria cause problems such as urinary-tract infections, some kinds of pneumonia, ear infections, pertussis (whooping cough), chlamydia, gonorrhea, and syphilis.
Protists – Protists are single-celled eukaryotes that cause diseases such as malaria and amoebic dysentery.
Animals –Common animal pathogens include lice, many types of worms, and parasitic wasps.
Prediction 3: In sexually reproducing populations, individuals choose mates that maximize diversity in their offspring
If there is a fitness advantage to diversity, parents can best maximize their offspring’s potential (and have more grand-offspring) with careful mate choice. There are numerous examples of organisms preferring mates that increase offspring diversity, and shunning mates that might do the opposite. Even many hermaphrodites, with both male and female sex organs, seek other hermaphrodites for copulation…even if they are capable of self-fertilization.
An excellent model for studying mate choice is Atlantic Salmon, an important commercial fish that lives its life in the ocean and returns to freshwaters to mate (or spawn ). Sofia Consuegra and Carlos Garcia de Leaniz compared offspring diversity of salmon that were mated in a commercial fish hatchery (and unable to choose their mates) against that of salmon allowed to choose mates in the wild. The hatchery-spawned fish exhibited lower diversity than did the wild-spawned fish. Furthermore, hatchery-spawned fish displayed a greater number of roundworm parasites ( Anisakis ) then did their wild-spawned counterparts (Figure 5). These results support the prediction that individuals will choose mates that maximize diversity in their offspring. Also, this work adds fuel to The Red Queen hypothesis by illustrating a potential benefit to Atlantic Salmon–namely, parasite avoidance.
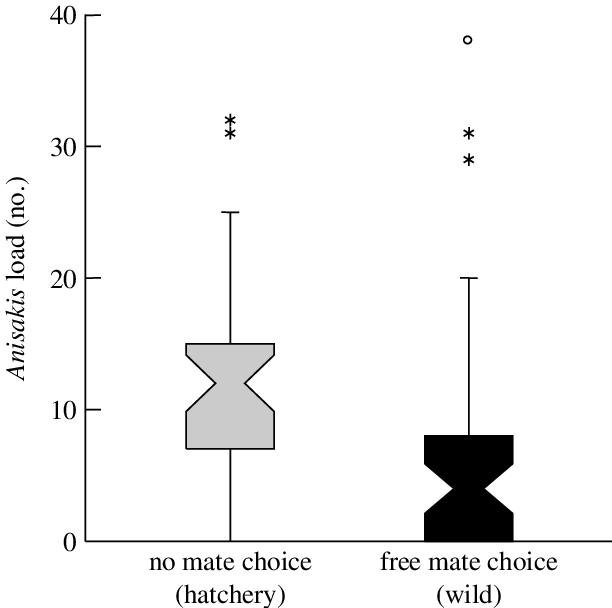
Introductory Biology: Evolutionary and Ecological Perspectives Copyright © by Various Authors - See Each Chapter Attribution is licensed under a Creative Commons Attribution 4.0 International License , except where otherwise noted.
Share This Book
Thank you for visiting nature.com. You are using a browser version with limited support for CSS. To obtain the best experience, we recommend you use a more up to date browser (or turn off compatibility mode in Internet Explorer). In the meantime, to ensure continued support, we are displaying the site without styles and JavaScript.
- View all journals
- Explore content
- About the journal
- Publish with us
- Sign up for alerts
- Published: 09 December 2009
Phylogenies reveal new interpretation of speciation and the Red Queen
- Chris Venditti 1 ,
- Andrew Meade 1 &
- Mark Pagel 1 , 2
Nature volume 463 , pages 349–352 ( 2010 ) Cite this article
4278 Accesses
113 Citations
40 Altmetric
Metrics details
The Red Queen 1 describes a view of nature in which species continually evolve but do not become better adapted. It is one of the more distinctive metaphors of evolutionary biology, but no test of its claim that speciation occurs at a constant rate 2 has ever been made against competing models that can predict virtually identical outcomes, nor has any mechanism been proposed that could cause the constant-rate phenomenon. Here we use 101 phylogenies of animal, plant and fungal taxa to test the constant-rate claim against four competing models. Phylogenetic branch lengths record the amount of time or evolutionary change between successive events of speciation. The models predict the distribution of these lengths by specifying how factors combine to bring about speciation, or by describing how rates of speciation vary throughout a tree. We find that the hypotheses that speciation follows the accumulation of many small events that act either multiplicatively or additively found support in 8% and none of the trees, respectively. A further 8% of trees hinted that the probability of speciation changes according to the amount of divergence from the ancestral species, and 6% suggested speciation rates vary among taxa. By comparison, 78% of the trees fit the simplest model in which new species emerge from single events, each rare but individually sufficient to cause speciation. This model predicts a constant rate of speciation, and provides a new interpretation of the Red Queen: the metaphor of species losing a race against a deteriorating environment is replaced by a view linking speciation to rare stochastic events that cause reproductive isolation. Attempts to understand species-radiations 3 or why some groups have more or fewer species should look to the size of the catalogue of potential causes of speciation shared by a group of closely related organisms rather than to how those causes combine.
This is a preview of subscription content, access via your institution
Access options
Subscribe to this journal
Receive 51 print issues and online access
185,98 € per year
only 3,65 € per issue
Rent or buy this article
Prices vary by article type
Prices may be subject to local taxes which are calculated during checkout
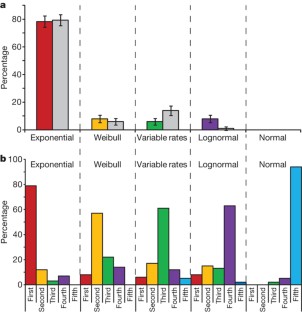
Similar content being viewed by others
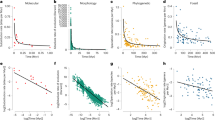
Conceptual and empirical bridges between micro- and macroevolution
Jonathan Rolland, L. Francisco Henao-Diaz, … Dolph Schluter
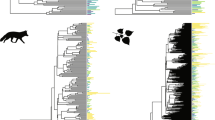
Arrested diversification? The phylogenetic distribution of poorly-diversifying lineages
Fernanda S. Caron & Marcio R. Pie
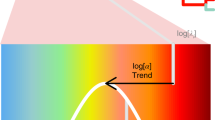
A model with many small shifts for estimating species-specific diversification rates
Odile Maliet, Florian Hartig & Hélène Morlon
Van Valen, L. A new evolutionary law. Evol. Theory 1 , 1–30 (1973)
Google Scholar
Stenseth, N. C. & Maynard Smith, J. Coevolution in ecosystems: Red Queen evolution or stasis? Evolution 38 , 870–880 (1984)
Article Google Scholar
Rabosky, D. L. & Lovette, I. J. Explosive evolutionary radiations: decreasing speciation or increasing extinction through time? Evolution 62 , 1866–1875 (2008)
Benton, M. J. in Palaeobiology: A Synthesis (eds Briggs, D. E. G. & Crowther, P. R.) 119–124 (Blackwells, 1990)
Pearson, P. N. Survivorship analysis of fossil taxa when real-time extinction rates vary: the Paleogene planktonic foraminifera. Paleobiology 18 , 115–131 (1992)
Nee, S., Mooers, A. O. & Harvey, P. H. Tempo and mode of evolution revealed from molecular phylogenies. Proc. Natl Acad. Sci. USA 89 , 8322–8326 (1992)
Article ADS CAS Google Scholar
Nee, S. Birth-death models in macroevolution. Annu. Rev. Ecol. Evol. Syst. 37 , 1–17 (2006)
Pagel, M. & Meade, A. A phylogenetic mixture model for detecting pattern-heterogeneity in gene sequence or character-state data. Syst. Biol. 53 , 571–581 (2004)
Venditti, C., Meade, A. & Pagel, M. Phylogenetic mixture models can reduce node-density artifacts. Syst. Biol. 57 , 286–293 (2008)
Venditti, C., Meade, A. & Pagel, M. Detecting the node-density artifact in phylogeny reconstruction. Syst. Biol. 55 , 637–643 (2006)
Pagel, M., Venditti, C. & Meade, A. Large punctuational contribution of speciation to evolutionary divergence at the molecular level. Science 314 , 119–121 (2006)
Gillespie, D. J. H. The Causes of Molecular Evolution (Oxford Univ. Press, 1991)
Pagel, M., Meade, A. & Scott, D. Assembly rules for protein networks derived from phylogenetic-statistical analysis of whole genomes. BMC Evol. Biol. 7 , S16 (2007)
Pagel, M. & Meade, A. Bayesian analysis of correlated evolution of discrete characters by reversible-jump Markov chain Monte Carlo. Am. Nat. 167 , 808–825 (2006)
Raftery, A. E. in Markov Chain Monte Carlo in Practice (eds Gilks, W. R., Richardson, S. & Spiegelhalter, D. J.) 145–161 (Chapman & Hall, 1996)
Colless, D. H. Phylogenetics: the theory and practice of phylogenetic systematics II (book review). Syst. Zool. 31 , 100–104 (1982)
Yule, G. U. A mathematical theory of evolution, based on the conclusions of Dr. JC Willis, FRS. Phil. Trans. R. Soc. Lond. B. 213 , 21–87 (1925)
Article ADS Google Scholar
Smith, S. A. & Donoghue, M. J. Rates of molecular evolution are linked to life history in flowering plants. Science 322 , 86–89 (2008)
Gillespie, J. H. The molecular clock may be an episodic clock. Proc. Natl Acad. Sci. USA 81 , 8009–8013 (1984)
Coyne, J. A. & Orr, H. A. Speciation 411–442 (Sinauer Associates, 2004)
Soltis, D. E., Soltis, P. S. & Tate, J. A. Advances in the study of polyploidy since plant speciation. New Phytol. 161 , 173–191 (2003)
Mitsainas, G. P., Rovatsos, M. T. H., Rizou, E. I. & Giagia-Athanasopoulou, E. Sex chromosome variability outlines the pathway to the chromosomal evolution in Microtus thomasi (Rodentia, Arvicolinae). Biol. J. Linn. Soc. 96 , 685–695 (2009)
Navarro, A. & Barton, N. H. Chromosomal speciation and molecular divergence — accelerated evolution in rearranged chromosomes. Science 300 , 321–324 (2003)
Orr, H. A. & Turelli, M. The evolution of postzygotic isolation: accumulating Dobzhansky-Muller incompatibilities. Evolution 55 , 1085–1094 (2001)
Article CAS Google Scholar
Seehausen, O. et al. Speciation through sensory drive in cichlid fish. Nature 455 , 620–626 (2008)
Mallet, J. Hybrid speciation. Nature 446 , 279–283 (2007)
Green, P. J. Reversible jump Markov chain Monte Carlo computation and Bayesian model determination. Biome 82 , 711–732 (1995)
MathSciNet MATH Google Scholar
Castoe, T. A. & Parkinson, C. L. Bayesian mixed models and the phylogeny of pitvipers (Viperidae: Serpentes). Mol. Phylogenet. Evol. 39 , 91–110 (2006)
Cameron, S. A., Hines, H. M. & Williams, P. H. A comprehensive phylogeny of the bumble bees ( Bombus ). Biol. J. Linn. Soc. 91 , 161–188 (2007)
Fritsch, P. W., Cruz, B. C., Almeda, F., Wang, Y. & Shi, S. Phylogeny of Symplocos based on DNA sequences of the chloroplast trnC-trnD intergenic region. Syst. Bot. 31 , 181–192 (2006)
Download references
Acknowledgements
We thank the Centre for Advanced Computing and Emerging Technologies (ACET) at the University of Reading for the use of the ThamesBlue supercomputer. We thank M. Turelli for calling our attention to Gillespie’s Poisson process model and for comments on earlier drafts of the paper. M. Steel pointed out the geometric distribution proof given in the Supplementary Information . This research was supported by grants to M.P. from the Natural Environment Research Council (NERC), UK, and the Leverhulme Trust.
Author Contributions C.V., A.M. and M.P. contributed to all aspects of this work.
Author information
Authors and affiliations.
School of Biological Sciences, University of Reading, Reading, Berkshire, RG6 6BX, UK ,
Chris Venditti, Andrew Meade & Mark Pagel
Santa Fe Institute, 1399 Hyde Park Road, Santa Fe, New Mexico 87501, USA ,
You can also search for this author in PubMed Google Scholar
Corresponding author
Correspondence to Mark Pagel .
Supplementary information
Supplementary information.
This file contains Supplementary Methods, Supplementary Data, Supplementary Figure S1 with Legend, Supplementary Tables S1-S3, a Supplementary List for Dataset sources and Supplementary References. (PDF 806 kb)
PowerPoint slides
Powerpoint slide for fig. 1, powerpoint slide for fig. 2, powerpoint slide for fig. 3, rights and permissions.
Reprints and permissions
About this article
Cite this article.
Venditti, C., Meade, A. & Pagel, M. Phylogenies reveal new interpretation of speciation and the Red Queen. Nature 463 , 349–352 (2010). https://doi.org/10.1038/nature08630
Download citation
Received : 18 August 2009
Accepted : 29 October 2009
Published : 09 December 2009
Issue Date : 21 January 2010
DOI : https://doi.org/10.1038/nature08630
Share this article
Anyone you share the following link with will be able to read this content:
Sorry, a shareable link is not currently available for this article.
Provided by the Springer Nature SharedIt content-sharing initiative
This article is cited by
4xtrifolium ambiguum and 2xt. occidentale hybridise despite wide geographic separation and polyploidisation: implications for clover breeding.
- W. M. Williams
- I. M. Verry
- N. W. Ellison
Theoretical and Applied Genetics (2019)
Dinosaurs reveal the geographical signature of an evolutionary radiation
- Ciara O’Donovan
- Andrew Meade
- Chris Venditti
Nature Ecology & Evolution (2018)
How Future Depends on Past and Rare Events in Systems of Life
- Giuseppe Longo
Foundations of Science (2018)
Speciation: Goldschmidt’s Chromosomal Heresy, Once Supported by Gould and Dawkins, is Again Reinstated
- Donald R. Forsdyke
Biological Theory (2017)
PhyInformR: phylogenetic experimental design and phylogenomic data exploration in R
- Alex Dornburg
- J. Nick Fisk
- Jeffrey P. Townsend
BMC Evolutionary Biology (2016)
By submitting a comment you agree to abide by our Terms and Community Guidelines . If you find something abusive or that does not comply with our terms or guidelines please flag it as inappropriate.
Quick links
- Explore articles by subject
- Guide to authors
- Editorial policies
Sign up for the Nature Briefing newsletter — what matters in science, free to your inbox daily.

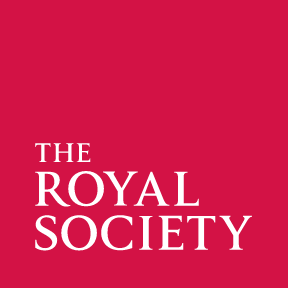
This year's Darwin Review: Revisiting the Red Queen
The red queen hypothesis states that in the battle for resources, species must continuously evolve just to keep up with their enemies, who themselves also evolve in response. this year, we revisit the seminal theory..
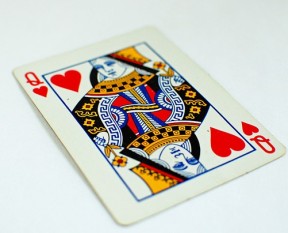
Proceedings B Darwin Reviews are special reviews published up to once a year. The aim of the Darwin Review is to showcase ideas and/or a field in biological science that is of very high interest to the whole diverse readership of Proceedings B, often being of particular relevance to strategic growth areas, importance to policy makers and/or having a bearing on the public that fund our science.
This year our Darwin review revisits a seminal theory in evolutionary research, Van Vaalen’s Red Queen Hypothesis. 40 years after its initial proposal the Red Queen is still informing research. Here the authors discuss their review and why now was the right time to highlight the Red Queen’s enduring legacy. You can read the full article here . Our previous Darwin review was on evolutionary medicine and can be found here .
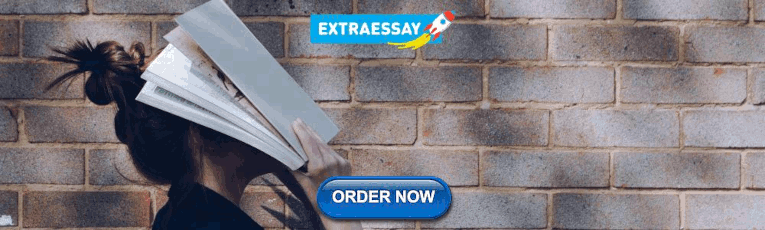
Tell us about the Red Queen hypothesis.
The Red Queen hypothesis was proposed over 40 years ago by the late evolutionary biologist Leigh Van Valen. It advanced evolutionary thinking beyond the idea that organisms were merely matched to their physical environment by suggesting that interactions between species (such as between hosts and parasites, predators and prey) would also be important in driving evolutionary change. In essence, the Red Queen hypothesis states that in the battle for resources, species must continuously evolve just to keep up with their enemies, who themselves also evolve in response. The result is that species constantly change but, relative to their enemies, don’t actually get any fitter – like running on an evolutionary treadmill. The name for the theory came from Lewis Carroll’s ‘Through the Looking Glass’ (aka Alice in Wonderland). Alice finds herself in a race with the Red Queen, and despite running as fast as she can, Alice stays in the same place. The Red Queen hypothesis, doubtless partly due to this imaginative metaphor, has become one of the most influential ideas in evolution.
How has the theory influenced evolutionary biology research since its original proposal?
Van Valen was interested in macroevolution, that is speciation and extinction, and used the Red Queen hypothesis to explain the apparently constant rates of extinction observed in the fossil record. And although this pattern has subsequently been challenged, Red Queen thinking is still important in macroevolution in terms of understanding the biological, as opposed to environmental, causes of diversification and extinction. In the 1980s, the utility of the Red Queen concept was rediscovered as a way of explaining the ubiquity of sexual reproduction in nature. The idea starts by considering that hosts and parasites are evolving together. If the hosts are sexual females, they can produce offspring that are genetically diverse and therefore avoid parasite infection. However, if hosts are clonal females, their offspring lack the ability to be as genetically diverse and will be more susceptible to attack by parasites. Thus, coevolution with parasites prevents the clones from taking over and gives sexual species an advantage. Increasingly, biologists think of the Red Queen hypothesis, not only as an explanation for sex, but also as a means of explaining rapid evolutionary change in hosts and their parasites in general.
What prompted you to write this review?
The adoption of the Red Queen as an explanation for the evolution and widespread maintenance of sex led to a narrowing of the definition of the Red Queen hypothesis. This meant that the far richer perspective of Van Valen’s original conceptual leap was in danger of being lost. To some extent, we wrote the review because we believed it was necessary to reemphasize a broader importance of the Red Queen hypothesis because it offers a powerful way in which to understand natural communities, how they evolve, and how they work. It is an important and useful idea. We were also inspired by the data emerging from new approaches of studying species interactions, including genome sequencing and experimental evolution in the laboratory. These present a more complex and nuanced picture of the effect of species interactions on evolution that is entirely consistent with a Red Queen view of nature. Additionally, we were aware that much of theory developed to understand how conflicts between species evolve could be usefully applied to understand conflicts within species. A variety of conflicts have been defined in this context: intragenomic conflicts (e.g. over the representation of chromosomes in gametes during meiosis), sexual conflicts (e.g. between males and females over reproductive effort and timing) and parent / offspring conflicts (e.g. over the allocation of resources to progeny). However, the evolutionary dynamics of these conflicts has never formally been synthesized within the Red Queen concept, despite the clear ability for these conflicts to generate the type of ‘running to stay still’ evolutionary dynamics. One of our aims in this review was therefore also to attempt this synthesis.
What does the future hold the red queen hypothesis?
It will certainly be informative to see how useful and explanatory is the incorporation of conflicts within species into the Red Queen framework. Beyond this, we recognise that much of our appreciation of the Red Queen currently comes from studying binary relationships (one parasite in one host). Extensions of this to more realistic scenarios, by incorporating parasites that evolve with a range of hosts, and hosts that evolve with a range of parasites, are therefore needed. How these will affect the speed of the Red Queen needs to be determined. Finally, there has been increasing use of comparative genomics to examine evolutionary dynamics. However, these analyses are limited in that the function of many genes is not known, making it difficult to determine the contribution of the Red Queen. Commonly, for instance, expression patterns indicate that specific genes are involved in male-female interactions, and could therefore be subject to coevolution. However, at the moment, we cannot differentiate the subset of genes that are involved with the male-female interface that are likely to be subject to Red Queen forces from those involved in a variety of other sex-specific physiological functions. We also have incomplete ascertainment of genes at the host-pathogen boundary: we understand host genes that are generically involved in defence much better than those which alter microbial invasion into a cell. We expect the results of comparative genomic analysis of Red Queen type processes to be made sharper as our understanding of gene function improves.
Meet the authors
Michael Brockhurst is Professor of Evolutionary Biology and a 50th Anniversary Chair at the University of York. He uses experimental evolution approaches to study the coevolution of species interactions with a particular focus on bacteria and their viral and genetic parasites. He is interested in the applied consequences of rapid microbial evolution in natural communities especially in clinically important pathogenic microbes.
Greg Hurst is Professor of Evolutionary Biology at the University of Liverpool. His main goal is to determine the ecological and evolutionary importance of heritable symbionts of insects (where symbiont is broadly defined and includes parasitism), and has an interest intragenomic and intra-specific conflicts more generally. His study animals include Drosophila, Nasonia wasps, butterflies and ladybirds, and the symbionts studied are largely microbial.
Kayla King is an Associate Professor at the University of Oxford. Her research explores the ecology and evolution of species interactions to ask fundamental questions about the maintenance of genetic and community-level diversity, sexual reproduction, and rapid evolutionary change. She focuses on interactions between hosts and their parasites as well as their microbiota.
Judith Mank is Professor of Evolutionary and Comparative Biology at University College London. She is interested in the constraints imposed on the genome by sexual conflict, and how selection navigates these restrictions of genome architecture to create intra-specific phenotypic diversity in the form of sexual dimorphism. She works on a range of study organisms, most recently birds, fish and flies.
Steve Paterson is Professor of Evolutionary Biology and a director of The Centre for Genomic Research at the University of Liverpool. He is primarily interested in understanding the forces that shape genetic diversity within host and parasite genomes. New genomic methods that allow us to address this question make it clear how much we owe to the Red Queen for our understanding of genome evolution and of the novel biology yet to be discovered from biotic interactions.
Tracey Chapman is Professor of Evolutionary Biology in the School of Biological Sciences at the University of East Anglia. She is interested in understanding the nature and the evolutionary potential of reproductive interactions between males and females. Such interactions are often subject to sexual conflict and can generate rapid evolutionary change.
Email updates
We promote excellence in science so that, together, we can benefit humanity and tackle the biggest challenges of our time.
Subscribe to our newsletters to be updated with the latest news on innovation, events, articles and reports.
What subscription are you interested in receiving? (Choose at least one subject)
- Subscriber Services
- For Authors
- Publications
- Archaeology
- Art & Architecture
- Bilingual dictionaries
- Classical studies
- Encyclopedias
- English Dictionaries and Thesauri
- Language reference
- Linguistics
- Media studies
- Medicine and health
- Names studies
- Performing arts
- Science and technology
- Social sciences
- Society and culture
- Overview Pages
- Subject Reference
- English Dictionaries
- Bilingual Dictionaries
Recently viewed (0)
- Save Search
- Share This Facebook LinkedIn Twitter
Related Content
Related overviews.
Romer's rule
environment
co-evolution
More Like This
Show all results sharing these subjects:
- Life Sciences
Red Queen hypothesis
Quick reference.
A hypothesis, proposed by L. M. Van Valen in the early 1970s, that describes how the coevolution of competing species creates a dynamic equilibrium, in which the probability of extinction remains fairly constant over time. Hence, evolution is seen neither as ‘progressive’ – with a species' chances of survival improving over time – nor as ‘escalatory’ – with increasing vulnerability to extinction over time. Instead, as one species evolves improvements that make it more competitive, its competitors experience selection pressures that force them to evolve in order to keep pace with it. Ones that lag too far behind will become extinct. The hypothesis is named after the remark made by the Red Queen in Lewis Carroll's Through the Looking Glass: “Here, you see, it takes all the running you can do, to keep in the same place.”
From: Red Queen hypothesis in A Dictionary of Biology »
Subjects: Science and technology — Life Sciences
Related content in Oxford Reference
Reference entries, red queen hypothesis, red queen hypothesis n..
View all reference entries »
View all related items in Oxford Reference »
Search for: 'Red Queen hypothesis' in Oxford Reference »
- Oxford University Press
PRINTED FROM OXFORD REFERENCE (www.oxfordreference.com). (c) Copyright Oxford University Press, 2023. All Rights Reserved. Under the terms of the licence agreement, an individual user may print out a PDF of a single entry from a reference work in OR for personal use (for details see Privacy Policy and Legal Notice ).
date: 13 April 2024
- Cookie Policy
- Privacy Policy
- Legal Notice
- Accessibility
- [66.249.64.20|195.190.12.77]
- 195.190.12.77
Character limit 500 /500
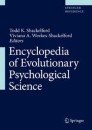
Encyclopedia of Evolutionary Psychological Science pp 6511–6512 Cite as
Red Queen Hypothesis, The
- Nicholas Primavera 3
- Reference work entry
- First Online: 01 January 2021
15 Accesses
Red Queen’s Race ; The Red Queen Effect
The Red Queen hypothesis is an evolutionary hypothesis that states that all living beings must constantly adjust, evolve, and reproduce while attempting to survive ever-evolving predators.
Introduction
This hypothesis was first proposed by Leigh Van Valen in 1973. The term “Red Queen” is a reference to a statement made by the Red Queen to Alice, characters in the popular 1871 novel Through the Looking-Glass , written by Lewis Carol.
The Red Queen Hypothesis and it’s Relevance
The statement that sparked this hypothesis is “Now, here , you see, it takes all the running you can do, to keep in the same place” (Carroll 1871 ). Van Valen’s reference is essentially a metaphor for an evolutionary arms race. Predators that undergo a beneficial adaption may spark a change in selection pressure when it comes to a group of prey. This, in turn, would continue in a positive feedback loop, which gives rise to a form of antagonistic coevolution....
This is a preview of subscription content, log in via an institution .
Carroll, L. (1991[1871]). 2: The garden of live flowers. In Through the looking-glass (The millennium fulcrum Edition 1.7 ed.).
Google Scholar
Gat, A. (2009). So why do people fight? Evolutionary theory and the causes of war. European Journal of International Relations, 15 (4), 571–599.
Article Google Scholar
Vermeij, G. J. (1987). Evolution and escalation. An ecological history of life (pp. 369–370). Princeton: Princeton University Press.
Book Google Scholar
Download references
Author information
Authors and affiliations.
SUNY New Paltz, New Paltz, NY, USA
Nicholas Primavera
You can also search for this author in PubMed Google Scholar
Corresponding author
Correspondence to Nicholas Primavera .
Editor information
Editors and affiliations.
Department of Psychology, Oakland University, Rochester, MI, USA
Todd K Shackelford
Viviana A Weekes-Shackelford
Section Editor information
University of Idaho, Moscow, ID, USA
Russell Jackson
Rights and permissions
Reprints and permissions
Copyright information
© 2021 Springer Nature Switzerland AG
About this entry
Cite this entry.
Primavera, N. (2021). Red Queen Hypothesis, The. In: Shackelford, T.K., Weekes-Shackelford, V.A. (eds) Encyclopedia of Evolutionary Psychological Science. Springer, Cham. https://doi.org/10.1007/978-3-319-19650-3_2663
Download citation
DOI : https://doi.org/10.1007/978-3-319-19650-3_2663
Published : 22 April 2021
Publisher Name : Springer, Cham
Print ISBN : 978-3-319-19649-7
Online ISBN : 978-3-319-19650-3
eBook Packages : Behavioral Science and Psychology Reference Module Humanities and Social Sciences Reference Module Business, Economics and Social Sciences
Share this entry
Anyone you share the following link with will be able to read this content:
Sorry, a shareable link is not currently available for this article.
Provided by the Springer Nature SharedIt content-sharing initiative
- Publish with us
Policies and ethics
- Find a journal
- Track your research
December 9, 2009
New species evolve in bursts
Red Queen hypothesis of gradual evolution undermined.
By Kerri Smith
New species might arise as a result of single rare events, rather than through the gradual accumulation of many small changes over time, according to a study of thousands of species and their evolutionary family trees.
This contradicts a widely accepted theory of how speciation occurs: that species are continually changing to keep pace with their environment, and that new species emerge as these changes accrue. Known as the 'Red Queen' hypothesis, it is named after the character in Lewis Carroll's book Through the Looking-Glass, and What Alice Found There who tells a surprised Alice: "Here, you see, it takes all the running you can do, to keep in the same place."
On supporting science journalism
If you're enjoying this article, consider supporting our award-winning journalism by subscribing . By purchasing a subscription you are helping to ensure the future of impactful stories about the discoveries and ideas shaping our world today.
The Red Queen hypothesis rests on the idea that species must continuously evolve just to hang on to their ecological niche. That gradual evolution is driven by the constant genetic churn of sexual selection.
A consequence of this is that all of the species in a particular family, or genus, gradually evolve to form new species at the same rate.
But Mark Pagel and his team at the University of Reading, UK, challenge this idea. In a paper published today in Nature, they compared four models of speciation -- one of which was the Red Queen hypothesis -- to see which best explains the rate of speciation in more than 100 species groups from the animal and plant kingdoms, including bumblebees, turtles, foxes and roses.
They looked at the lengths of branches in thousands of species' evolutionary trees contained within these groups to estimate the time periods between speciation events.
When the team compared how well the four models fitted the groups' evolutionary histories, the Red Queen idea that species form through a catalogue of incremental changes fitted no more than 8% of the family trees.
Conversely, almost 80% of the trees fitted a model in which new species emerge from single rare evolutionary events. The Red Queen, it seems, is not running to keep up, but jumping a longer distance and then pausing for a while1.
"What we've shown is that speciation is about happy accidents -- rare events that happen in the environment that cause a species to speciate," says Pagel. These events could include a mountain range being thrust up or a shift in climate, he says.
The team's findings might stir things up in the world of evolutionary biology. "It really goes against the grain because most of us have this Darwinian view of speciation," says Pagel. "What we're saying is that to think about natural selection as the cause of speciation is perhaps wrong."
Mike Benton, a palaeontologist at the University of Bristol, UK, agrees that the work might ruffle a few feathers, adding that it could also shift attention to how groups of species evolve, rather than the minutiae of competition or predation effects that affect a single species. Where speciation is concerned, at least, "maybe all of this squabbling in the undergrowth is quite irrelevant".
Evolutionary biologists will also "look closely at the methods", Benton predicts. The paper's heavily computational approach, crunching large amounts of data from phylogenetic trees, has not traditionally been applied to evolutionary theories.
But Pagel thinks they will be able to convince others that their approach is useful. "We think people will come around because it will start to unravel some mysteries about speciation," he says.
Getting somewhere with the Red Queen: chasing a biologically modern definition of the hypothesis
Affiliations.
- 1 Department of Ecology and Evolutionary Biology, University of Kansas, Lawrence, KS 66045, USA [email protected].
- 2 Biodiversity Institute, University of Kansas, Lawrence, KS 66045, USA.
- 3 Department of Ecology and Evolutionary Biology, University of Kansas, Lawrence, KS 66045, USA.
- PMID: 29720444
- PMCID: PMC6012711
- DOI: 10.1098/rsbl.2017.0734
The Red Queen hypothesis (RQH) is both familiar and murky, with a scope and range that has broadened beyond its original focus. Although originally developed in the palaeontological arena, it now encompasses many evolutionary theories that champion biotic interactions as significant mechanisms for evolutionary change. As such it de-emphasizes the important role of abiotic drivers in evolution, even though such a role is frequently posited to be pivotal. Concomitant with this shift in focus, several studies challenged the validity of the RQH and downplayed its propriety. Herein, we examine in detail the assumptions that underpin the RQH in the hopes of furthering conceptual understanding and promoting appropriate application of the hypothesis. We identify issues and inconsistencies with the assumptions of the RQH, and propose a redefinition where the Red Queen's reign is restricted to certain types of biotic interactions and evolutionary patterns occurring at the population level.
Keywords: Red Queen hypothesis; competition; environment; extinction; macroevolution.
© 2018 The Author(s).
Publication types
- Research Support, U.S. Gov't, Non-P.H.S.
- Biological Evolution*
- Competitive Behavior
- Extinction, Biological*
- Models, Biological
- Paleontology
- Selection, Genetic

An official website of the United States government
The .gov means it’s official. Federal government websites often end in .gov or .mil. Before sharing sensitive information, make sure you’re on a federal government site.
The site is secure. The https:// ensures that you are connecting to the official website and that any information you provide is encrypted and transmitted securely.
- Publications
- Account settings
Preview improvements coming to the PMC website in October 2024. Learn More or Try it out now .
- Advanced Search
- Journal List
- HHS Author Manuscripts

On the Causes of Selection for Recombination underlying the Red Queen Hypothesis
Marcel salathé.
1 Department of Biology, Stanford University, Stanford, CA 94305, USA
Roger D. Kouyos
2 Institute of Integrative Biology, ETH Zurich, CH-8092 Zurich, Switzerland
Sebastian Bonhoeffer
The vast majority of plant and animal species reproduce sexually despite the costs associated with sexual reproduction. Genetic recombination might outweigh these costs if it helps to escape parasite pressure by creating rare or novel genotypes, an idea known as the Red Queen Hypothesis. Selection for recombination can be driven by short-term and long-term effects, but the relative importance of these effects and their dependency on the parameters of an antagonistic species interaction remain unclear. We use computer simulations of a mathematical model of host-parasite coevolution to measure those effects under a wide range of parameters. We find that the real driving force underlying the Red Queen Hypothesis is neither the immediate, next generation short-term effect nor the long term effect, but in fact a delayed short-term effect. Our results highlight the importance to differentiate clearly between the immediate and the delayed short-term effect when attempting to elucidate the mechanism underlying selection for recombination in the Red Queen Hypothesis.
Introduction
Why is it that in almost all animal and plant species, offspring individuals have different combinations of genes from their parents? While the proximate cause of this phenomenon, genetic recombination, has been worked out in great detail, its ultimate cause continues to remain enigmatic (see Otto 2008, this issue). Numerous hypotheses have been proposed to explain the evolutionary maintenance of recombination. Because the only direct effect of genetic recombination is the reduction of statistical associations among genes (i.e., linkage disequilibria, LD), any hypothesis that aims to explain the selective benefit of recombination must explain (i) why breaking up genetic associations is beneficial, and (ii) by which process such genetic associations are continually recreated.
One possible benefit of recombination is that breaking up genetic associations may result in an increase in genetic variance, which may in turn increase the response to selection. The problem with this argument is that recombination only increases genetic variance when genotypes with intermediate fitness are over-represented in a population (or, in mathematical terms, when LD < 0). If the genotypes with high or low fitness are over-represented (i.e., LD > 0), recombination will result in a decrease of genetic variance, which in turn hampers the response to selection.
Two processes have been identified as potential causes of negative LD: negative epistasis among deleterious alleles, and drift in conjunction with selection. Negative epistasis among deleterious alleles implies that alleles have a stronger fitness reducing effect in combination than expected based on their effect in isolation. Whenever combinations of deleterious alleles are more unfit than expected (negative epistasis), it is intuitive that these combinations become less frequent following selection than expected on the basis of the frequency of the individual alleles. Hence, negative epistasis acts as a force that generates negative LD. Drift refers to the change of allele frequencies in finite populations due to chance events, and while drift can cause both negative and positive LD, positive LD disappear faster by selection than negative LD. The net result of this process is a prevalence of negative LD, an effect commonly known as the Hill-Robertson effect ( Hill & Robertson, 1966 ).
Speeding up selection by increasing variance is a process that takes some time: first, the associations must be broken down, releasing beneficial alleles from their deleterious backgrounds, and then those alleles must spread in the population, dragging alleles that increase rates of recombination with them. This effect, known as the long-term effect , however, is not the only effect of recombination ( Barton 1995 ). By breaking down genetic associations, recombination also has an effect that is immediate: it creates new combinations of alleles that might be more or less fit than the combinations of alleles in the previous generation. This effect is known as the short-term effect , because it becomes manifest immediately in the next generation. The effect is generally thought to be detrimental in systems at selection-mutation(-drift) balance. The reason is that the combinations of alleles that are overrepresented in a population have been favoured by selection in the past, and thus breaking them down typically results in a population whose average fitness is lower.
The net selective effect on any gene that influences the rate of recombination is thus a result of two effects, the short-term and the long-term effect. In models that are based on simple fitness landscapes that are constant over time, it is well understood under which circumstances the two effects lead to a net benefit for recombination (for a review, see Otto & Lenormand 2002 ). In such models, the short-term effect generally tends to be negative, because recombination breaks down the overly fit and thus overly frequent allele combinations. Provided the population is sufficiently large such that epistasis is the dominating force generating LD, the long-term effect is beneficial only when epistasis (and thus LD) is negative. The long-term effect can outweigh the short-term effect if epistasis is negative, but sufficiently weak.
The Red Queen hypothesis
The assumption that fitness landscapes are constant over time is overly simplistic for many biological scenarios. Thus an alternative hypothesis to explain the ubiquity of genetic recombination is that it may continually create novel genotypes that are at a selective advantage in an ever-changing environment. The idea that recombination might be beneficial in changing environments can already be found in the writings of Wright, Muller and others ( Wright 1932 ; Muller 1932 ; Sturtevant & Mather 1938 ; Haldane 1949 ). Initially, there was some doubt as to whether environments change fast enough to cause selection for recombination ( Charlesworth 1976 ; Bell 1982 ), but many theoretical studies have shown in the meanwhile that host-parasite coevolution, a process that likely affects almost all species, provides the necessary conditions for such a mechanism to work (for a review, see Salathé et al. 2008a ). As parasites adapt quickly to become better at infecting the most frequent host genotype, recombination in the host might be beneficial because it creates rare or novel genotypes that are at a selective advantage. This idea is nowadays known as the Red Queen hypothesis (RQH) ( Bell 1982 ).
It is worth revisiting the conditions under which the RQH works. First, the outcome of an interaction between a host and a parasite needs to be at least in part genetically determined, an assumption that is likely to be met frequently. Second, selection needs to be strong on at least one of the coevolving species ( Salathé et al. 2008b ). There has been considerable debate about whether parasites are generally virulent enough to cause enough selection on hosts for recombination to be beneficial ( Peters & Lively 1999 ; Schmid-Hempel & Jokela 2002 ; Otto & Nusimer 2004 ). However, it is plausible to assume that parasites often do experience substantial selection from the host, because many parasites cannot reproduce at all if they fail to infect a host (or reproduce at much slower rates outside of a host than within a host). Moreover, even if selection on the parasite was relatively weak, the fact that most parasites have much shorter generation times than their hosts implies that they can adapt much faster than their hosts, effectively increasing the selective pressure on hosts ( Salathé et al. 2008b ). Third, the interactions between host loci mediating parasite resistance need to be epistatic ( Kouyos et al. 2007 ) or display dominance ( Agrawal & Otto 2006 ). In the absence of epistatic interactions among loci, strong LD of constant sign build up quickly which cause strong selection against recombination ( Kouyos et al. 2008 ). Data from plant and animal studies suggest that epistatic interactions among disease resistance loci are indeed prevalent ( Kover & Caicedo 2001 ).
Thus, while theoretical studies have clarified the role of both the strength of selection and of the genetic interaction system necessary for the RQH to work, the exact causes of selection for recombination remain to be fully investigated. Three recent studies have shed some light on this issue. Peters & Lively (2007) investigated the spread of a gene for recombination in an initially non-recombining population and found that recombination provides an “immediate (next-generation) fitness benefit and a delayed (two or more generations) increase in the rate of response to directional selection”. Gandon & Otto (2007) analyzed a model where allele frequencies remain fixed over time (the so-called Nee model ( Nee 1989 )), which has the benefit that directional selection is absent, and a long-term effect based on increased genetic variance can thus be excluded. Their analysis focused on the time lag between epistasis and LD fluctuations, arguing that this lag yields a short-term benefit for recombination in generations where LD and epistasis have opposite sign. Finally, we argued ( Salathé et al. 2008a ) that the short-term effect should be subdivided into an immediate effect and a delayed effect. Using the Nee model, we demonstrated that the immediate short-term effect is often detrimental while the delayed short term-effect is generally beneficial for recombination. In particular, we argued that the immediate short-term effect, approximated by the product of epistasis and LD, has almost no predictive power on the evolution of recombination.
Disentangling the various effects affecting the selection pressure on a recombination modifier is important both theoretically and empirically. For example, if the immediate short-term effect in the next generation does not have any predictive power on the direction of selection for recombination, then measuring this effect will not be useful to demonstrate the role of the RQH in natural populations. Our previous analysis ( Salathé 2008a ) offered only limited insight into the mechanism underlying the selection for recombination in the RQH as it was based on the somewhat unrealistic Nee model, and was restricted to a small parameter space. In this study, we will extend our previous work by extending the parameter space and by presenting an approach that allows disentangling the short-term effect (both immediate and delayed) from the long-term effect in a model where both the short-term and the long-term effect are present. We find that neither the long-term effect nor the immediate short-term effect have much power in predicting whether recombination is favoured or disfavoured in Red Queen models. In fact, for most of the parameter space investigated, both effects are either irrelevant (i.e. of negligible magnitude) or quite often misleading (i.e. pointing in the opposite direction) if looked at in isolation. We identify the delayed short-term effect as the best predictor for the evolution of recombination in the RQH.
Material & Methods
We use a frequency-based deterministic model that has been described elsewhere to simulate host-parasite coevolution ( Kouyos et al. 2007 ). Briefly, hosts and parasites are haploid and have two loci with two possible alleles (0 and 1) that determine the outcome of an interaction between host and parasite, depending on the interaction model used (see below). Hosts possess an additional modifier locus with two possible alleles, m (wildtype) and M (mutant) that define the rate at which hosts recombine. The modifier locus is positioned at one end of the genome. Thus, there are 8 possible host haplotypes ( 00m , 00M , 01m , 01M , 10m , 10M , 11m and 11M ) and 4 possible parasite haplotypes ( 00 , 01 , 10 and 11 ).
A simulation runs for t time steps, which corresponds to t host or parasite generations. Hosts and parasites are assumed to have the same generation time. All results presented below are from simulations that ran for 2,000 host generations. At each time step, the following processes occur: host reproduction, host selection, host mutation, parasite reproduction, parasite selection, and parasite mutation. The recursion equations for hosts and parasite genotype frequencies, f H and f P , are as follows:
where the superscripts H and P denote the host and parasite populations, respectively, and the function RSM denotes the successive action of reproduction, selection and mutation. We now describe these three processes in detail.
We assume that parasites reproduce clonally while hosts reproduce by recombining their genotypes. Recombination occurs at a rate that depends on the alleles present at the modifier locus of two recombining genotypes. The recombination rate between the interaction loci is r baseline if both recombining genomes carry allele m . It is increased by a small amount Δ r or 2Δ r , respectively, if one or both genomes carry allele M . The recombination rate between the modifier and the interaction loci is r modifier (only one recombination rate has to be defined in this case, because a recombination event between the modifier locus and the interaction loci only has an effect if the modifier alleles of the two recombining genomes are different). Because we are interested in the fate of the allele M increasing the recombination rate, Δ r > 0 in all simulations.
Selection is determined by a fitness matrix as defined by the interaction model chosen (see below, and Salathé et al. 2008a , Box 1) and by the genotype frequencies of the coevolving species. More explicitly, the fitness matrices W H :=( w H ij ) 4×4 and W P :=( w P ij ) 4×4 define the fitness of each possible interaction of host genotype i and parasite genotype j in a two-locus/two-allele system. Assuming that the probability of interaction between host genotype i and parasite genotype j is the product of their respective frequencies, f H i and f P j , the frequency of host genotype i after selection, f' H i , is
where w H i is the fitness of host genotype i, given by
The parasite frequencies after selection, f' P , are calculated analogously.
Finally, mutations at the interaction loci occur at a rate of 10 −5 per locus per generation, both in hosts and parasites. If a mutation occurs, allele 0 becomes allele 1 and vice versa. There is no mutation at the modifier locus.
Interaction model
We use two different interaction models: the so-called Nee model, and the matching allele model (MA model). The MA model is widely used in the context of the RQH, and in the two-locus/two-allele setup used here, the MA model assumes that a host genotype is susceptible to a given parasite genotype if and only if the alleles at both interaction loci match. In that case, the fitness of the host genotype is 1- s H , and the fitness of the parasite genotype is 1. In all other cases (i.e. where the host and parasite genotype do not fully match), the host is assumed to be fully resistant, and thus the fitness of the host genotype is 1, and the fitness of the parasite genotype is 1- s P .
The Nee model assumes that the host genotype is resistant to a given parasite genotype if and only if the alleles at exactly one interaction locus match; for example, the host genotype 01 is resistant to parasite genotypes 11 and 00, but not to 01 and 10. In the case of resistance, the fitness of the host genotype is 1, and the fitness of the parasite genotype is 1- s P . In all other cases, the fitness of the host genotype is 1- s H , and the fitness of the parasite genotype is 1. The Nee model lacks biological realism, but has the useful property that the allele frequencies converge to 0.5. Constant allele frequencies imply that directional selection is absent, and therefore, after a transient burn-in phase, directional selection can be neglected, and thus there is no long-term effect selecting for recombination in this model.
Simulations
Both host and parasite populations are assumed to be infinite in size, and they are initiated with random genotype frequencies, except at the modifier locus where only allele m is initially present (i.e. recombination occurs initially at a rate r baseline ). In order to investigate the fate of the modifier allele ( M ) increasing recombination, we introduce the M allele in 0.01% of the population after a burn-in phase of 1,000 host generations. We then let the simulation run for another 1,000 host generations, during which we measure a variety of quantities such as epistasis, linkage disequilibrium, and the frequency of the modifier allele M .
The effect of altering linkage disequilibrium on the mean fitness of offspring depends on epistasis measured on an additive scale. Epistasis in the host population is thus measured as
where w H i is the fitness of host genotype i as defined in eq. 4 .
Linkage disequilibrium in the host is measured as
Measuring short-term and long-term effects
In order to disentangle the various effects of selection acting on a recombination modifier, we aim to quantify the short-term effect and the long-term effect on the recombination modifier allele M . The short-term effect acts in the generation immediately following a given recombination event ( Figure 1 , line A), but it is not limited only to that generation ( Figure 1 , line B). The long-term effect of a given recombination event only begins to act one generation after the event. In the generation immediately following the recombination event, genetic associations are broken down, but only in the subsequent generations does the increased (or decreased) variance have a selective effect on the modifier ( Figure 1 , line C).

Disentangling the effects of a recombination modifier. A recombination event is indicated by the thick dotted line. In the immediately following generation g 0 , only the immediate short-term effect (A) is causing selection for or against recombination. After that (g n>0 ), two types of effects can be responsible for selection: the delayed short-term effect (B) which is a continuation of the short-term effect, and the long-term effect (C) based on directional selection. Because (B) and (C) occur at the same time, it is important to distinguish between them clearly. The total short-term effect is given by lines A + B.
In the Nee model, where directional selection is absent, we can directly measure the short-term effect acting immediately in the next generation (the so-called immediate short-term effect) and the short-term effect acting after one generation (the so-called delayed short-term effect). In essence, we measure the short-term effect by comparing populations that differ in their recombination rates only in a single generation. More precisely we implement the following procedure: After each generation of a simulation, we pause the simulation and create four test populations (two host populations H1 and H2 , and two parasite populations P1 and P2 ), which we initiate with the current host and parasite frequencies of the main populations. We then let the test populations coevolve for 50 host generations ( H1 with P1 , and H2 with P2 ), with the only difference being that for the first generation in population H2 , Δ r is set to zero (i.e. the modifier has no effect on the recombination rate). After this first generation, Δ r is set back to its original value. We then measure selection acting on the modifier n generations after the first generation ( s n ) as the difference between the mean fitness of the genotypes carrying allele M in populations H1 and H2 , divided by the total mean fitness, in the ( n + 1 )th generation after the initiation of the test populations:
The value of s 0 corresponds to the immediate short-term effect, while the sum of all s n values where n > 0 corresponds to the delayed short-term effect.
In the MA model, we use the same procedure to measure the immediate short-term effect, but because the delayed short-term effect and the long-term effect act at the same time, it is not possible to differentiate between these two effects with the method described above. Instead, we use a mathematical approximation to calculate the strength of selection acting on the modifier due to short-term and long-term effects. The short-term effect is due to differences in LD of the M -linked and m -linked genotypes, while the long-term effect is due to differences in allele frequencies of the M -linked and m -linked genotypes. Mathematically, the strength of selection on the modifier can be expressed as
where w ̅ M and w ̅ m denote the mean fitness of genotypes with modifier allele M and m , respectively. The mean fitness is a function of the allele frequencies and LD, i.e. w ̅ = w ̅ ( p 1 , p 2 , LD ), where p i denotes the allele frequency at interaction locus i . For a weak modifier, s M can be approximated as
where p i M and D M denote allele frequencies and LD of the subpopulation with allele M , and p i m and D m denote allele frequencies and LD of the subpopulation with allele m . The first two terms correspond to the allele-frequency-dependent component of s M , whereas the third term corresponds to the LD-dependent component of s M . Hence
(See appendix for explicit expressions of the short- and long-term effect).
Our first goal was to extend our previous analysis of the short-term effect in the Nee model where, due to the absence of directional selection, there is no long-term effect. We have previously argued that although the short-term effect is manifest immediately in the next generation, it is also manifest in subsequent generations (i.e., with a delay ). Moreover, we showed that the immediate short-term effect can select against recombination, while the delayed short-term effect selects for recombination ( Salathé et al. 2008a ). In particular, the immediate short-term effect is usually much weaker than the delayed short-term effect and has thus almost no predictive power for the evolution of recombination. Figure 2 extends those results into the entire parameter space of possible selection strengths acting on both host ( s H , i.e. virulence) and parasite ( s P ).

Results from the Nee model. The following colour-coding was used: red to yellow: selection for recombination; blue to black: selection against recombination. (A) Change of modifier frequency, (B) LD x epistasis, (C) immediate short-term effect, (D) delayed short-term effect. The short-term effects are measured as described in the main text (see “Material & Methods”). Parameters used: r baseline = 0.0025; Δ r = 0.0005; r modifier = 0.5.
Figure 2a shows selection on a modifier causing increased recombination. Such a modifier is selected when at least one of the coevolving species experiences sufficient selection, confirming similar findings in the matching allele model ( Salathé at al. 2008b ). As expected, the product of LD and epistasis ( fig. 2b ) and the immediate short-term effect ( fig. 2c ) are qualitatively equivalent but opposite in sign: a negative product corresponds to a positive immediate short-term effect and vice versa. However, the immediate short-term effect ( fig. 2c ) is weak compared to the effective selection on the modifier ( fig. 2a ), in stark contrast to the delayed short-term effect which is much stronger ( fig. 2d ). It is the sum of the immediate and the delayed short-term effect that is acting on the modifier, but as can be seen from figs. 2c and 2d , the immediate effect is largely irrelevant quantitatively, and can be misleading qualitatively.
Epistasis and LD for a certain time span of a typical simulation run are shown in Figure 3a . Figure 3b shows the short-term effect measured with a delay of 0 (solid line), 1 (dashed lines) and 2 (dotted line) generations. Thus, the solid line shows the immediate short-term effect ( n = 0). Delayed short-term effects ( n > 0 ) show positive selection more frequently than the immediate effect. The reason for this is that the immediate and the delayed short-term effect become negative at the same time, but the delayed short-term effect becomes positive earlier than the immediate short-term effect (around time step 8 and 24 in Figure 3b ). Why is this so? The delayed effect depends on “future” epistasis in the sense that the combination of alleles created now will not only experience natural selection on the current fitness landscape, but also on the fitness landscape present in n generations. Thus, starting from a given value of LD at generation t , the immediate and delayed short-term effect can be qualitatively different only if the sign of epistasis at generation t + n is different from the sign of epistasis at generation t . As can be seen in Figure 3a , epistasis changes its sign only after a period during which epistasis and LD were of the same sign (i.e. during which the immediate short-term effect selected against recombination). Consequently, the two effects can be qualitatively different only when the immediate short-term effect is negative (selecting against recombination), and the delayed short-term effect is positive.

Typical dynamics of the Nee model. (A) LD (solid line) and epistasis (dashed line) of host population. (B) immediate short-term effect ( s 0 ) and the delayed short-term effect s 1 and s 2 . As an example of the immediate and delayed short-term effect pointing in different direction, consider time step 9 where the s 0 < 0 and s 2 > 0. The arrows point to the current and future epistasis experienced by the host population as present at time step 9 (see main text). Parameters used: s H = 0.2, s P = 1; r baseline = 0.0025; Δ r = 0.0005; r modifier = 0.5.
We now turn our attention to the matching allele model. In contrast to the Nee model, the matching allele model exhibits directional selection and thus a potential for a long-term effect. We find that the immediate short-term effect ( figs. 4c & 5c ) is generally detrimental to recombination, in strong qualitative contrast to the observed selection on the modifier ( figs. 4a & 5a ). It is, however, quantitatively very weak and can thus be safely ignored. These observations are independent of how strongly the modifier is linked to the interaction loci. Interestingly, the only region where the immediate short-term effect is appreciably strong is when s P ≈ 1, which is consistent with previous findings ( Peters & Lively, 2007 ).

Results from the matching allele model. (A) Change of modifier frequency, (B) long-term effect, (C) immediate short-term effect (measured as in fig. 2C ), (D) total short-term effect as calculated by equation 10 , (E) sum of total short-term effect and long term effect as calculated by equation 10 , (F) delayed short-term effect, given by (D) – (C). Parameters: r baseline = 0.0025; Δ r = 0.0005; r modifier = r baseline . Colour-coding as in Figure 2 .

Same settings as in Figure 4 , but with a completely unlinked modifier locus, i.e r modifier = 0.5. Colour-coding as in Figure 2 .
In contrast, the delayed short-term effect ( figs. 4f & 5f ) is generally in good agreement with selection on the modifier. Again, this observation is largely independent of how strongly the modifier is linked to the interaction loci.
Finally, the long-term effect and the delayed short-term effect are of about the same magnitude, but the direction of the long-term effect depends strongly on the linkage between modifier and the interaction loci. When that linkage is very strong, the long-term effect is detrimental to recombination in areas of moderate selection and beneficial to recombination in areas of strong selection ( fig. 4b ). When the modifier is loosely linked, the opposite pattern is observed: the long-term effect is beneficial to recombination in areas of weak selection and detrimental to recombination in areas of strong selection ( fig. 5b ).
Taken together, these observations suggest that in general, the delayed short-term effect is the best qualitative predictor for selection of recombination. The long-term effect can support the delayed short-term effect, but even if it causes selection against recombination, it is hardly ever strong enough to override the effect of the delayed short-term effect. This is particularly apparent in the case of a completely unlinked modifier, where the long-term effect and the delayed short-term effect result in almost completely opposite selection pressures, with the long-term effect pointing in the wrong direction over almost the entire parameter space.
Overall, our simulations suggest that the real driving force underlying the RQH is neither the immediate short-term effect nor the long-term effect, but in fact the delayed short-term effect. As always, things turn out to be more complicated when considering greater levels of detail, but in general, this statement holds, at least within the realm of the simulations presented here.
In the Nee model, where a long-term effect can be excluded, the delayed short-term effect is a perfect qualitative predictor for the evolution of recombination. In terms of quantitative prediction, the contribution of the delayed short-term effect is about an order of magnitude higher than the immediate short-term effect. As we he have argued previously ( Salathé et al. 2008a ), equating the short-term effect with the immediate short-term effect only is therefore problematic. As can be seen clearly in Figure 2c , the immediate short-term effect would predict selection against higher levels of recombination in more than half of the parameter space, while higher recombination is in fact selected for in more than 90% of the parameter space.
Why is it that the immediate short-term effect is not a good predictor for selection of recombination? It is worth remembering that the short-term effect is the effect by which recombination creates new combinations of alleles that might be more or less fit than the combinations of alleles in the previous generation. While this effect becomes manifest immediately in the next generation, it is not confined to that generation only. A modifier associated with new allelic combinations created by recombination will also be subject to selection in future generations, i.e. with a delay. This delay is a fundamentally important idiosyncrasy of the RQH: because of fluctuating epistasis, the combinations of alleles that are unfit (or fit) in the current generation might be fit (or unfit) in future generations. Figure 3b shows that the effect in future generations is, on average, more positive (dashed and dotted line) than the effect in the first generation of recombinant offspring (solid line). Clearly, the delayed effects become less important as the linkage between the modifier and the interaction loci becomes weaker. We have shown previously, however, that even in the case of minimal linkage (i.e. full recombination between the modifier and the interaction loci), the delayed effects outweigh the immediate effect (see fig. 1b , Box 2, in Salathé 2008a ).
The results of the matching allele model show that the discrepancy between immediate and delayed short-term effect is even more pronounced. Independent of the linkage between modifier and interaction loci, the immediate short-term effect causes selection against recombination across almost the entire parameter space ( fig. 4c & 5c ), while the delayed short-term effect causes selection for recombination. However, because the immediate short-term effect is one to two orders of magnitude weaker than the delayed short-term effect, it is also largely irrelevant in determining the fate of a recombination modifier. The delayed short-term effect appears to be a very good qualitative proxy for selection on the modifier.
The long-term effect is approximately of the same magnitude as the delayed short-term effect. However, the pattern of the long-term effect depends strongly on the linkage between modifier and interaction loci. When this linkage is strong ( fig. 4 ), the long-term effect causes selection for recombination when at least one of the coevolving species experiences sufficient selection from their interaction (with the exception of very weak selection on both host and parasite). However, when linkage is loose ( fig. 5 ), the opposite pattern emerges: the long-term effect then causes selection against recombination when at least one of the coevolving species experiences sufficient selection from their interaction (with the notable exception of very strong selection on both host and parasite). Thus, in the case of loose linkage between modifier and interaction loci, the long-term effect and the delayed short-term effect exhibit roughly opposite patterns, but because the delayed short-term effect is slightly stronger in this case, the qualitative outcome on selection on the modifier is predicted better by the delayed short-term effect.
At first sight, it might seem that while the delayed short-term effect is a better proxy for the evolution of recombination than the long-term effect, its superiority is only marginal. However, this outcome strongly depends on which area in the parameter space one is looking at. Little is known about the effective fitness effects of parasites on hosts and vice versa, but while there are some parasites that have devastating effects on the fitness of their hosts (castrating parasites, for example), the majority of parasites probably exert much weaker selective pressure. If we zoom in on the area where s H <= 0.2, a clearer picture emerges: the long-term effect and the delayed short-term effect are almost in perfect disagreement about the direction of selection they cause on the modifier, independent of the linkage between modifier and interaction loci. The actual direction of selection on the modifier, however, is always in agreement with the delayed short-term effect: in no case is the long-term effect strong enough to reverse the selection caused by the delayed short-term effect.
Our results are limited in three important ways. First, the results obtained in this study are based on two specific interaction models (the Nee model and the matching allele model). To what extent these results hold in other models remains to be investigated. In the matching allele model, for example, the long-term effect is only of slightly weaker magnitude than the delayed short-term effect. Thus, it could very well be that a small departure from the strict matching allele model would affect the slightly skewed balance between the two effects and eventually reverse it. Second, we have focussed exclusively on the spread of a recombination modifier. Recent work ( Peters & Lively 2007 ) has shown that the short- and long-term effects during the spread of a modifier can be very different from the effects at equilibrium. Finally, our results are based on relatively low recombination rates, an assumption that decreases the parameter regions where the conditions for quasi-linkage equilibrium (QLE) are met (i.e., weak selection relative to recombination). For example, Otto & Nuismer have shown (2004) that the long-term effect selects for recombination at QLE, indicating that the parameter region in figs. 4b and and5b 5b (where the long-term effect selects for higher recombination near the origin) would expand.
A continuing problem is that the terminology of short-term and long-term effects is potentially confusing, in particular in the context of the RQH. The short-term effect was named to reflect the fact that it already becomes manifest in the generation immediately following a recombination event. But since the short-term effect can also be felt many generations later (i.e. in the long-term), it can easily be confused with the long-term effect. At the same time, the short-term effect can also be confused with an effect restricted to the next generation only, which can be misleading too because, as we have shown here, the effect in the generation following a recombination event (the immediate short-term effect) can select against recombination while the effect in subsequent generations (the delayed short-term effect) can select for recombination. In practice, this also means that measuring the recombination load, given here by the immediate short term effect, will not be sufficient to asses the validity of the RQH. Because the delayed short-term effect turns out to be crucial for the evolution of recombination in the RQH - at least in the interaction models considered here - ignoring this effect can lead to the wrong conclusions. Our results therefore highlight the importance of differentiating clearly between the immediate and the delayed short-term effect when attempting to elucidate the mechanism underlying selection for recombination in the RQH.
Acknowledgements
We would like to thank Sally Otto for the invitation to the 2008 Vice President Symposium of American Society of Naturalists. Thanks also to Curt Lively, Dennis Roze and Rahel Salathé for helpful comments on the manuscript. This work was supported by the Swiss National Science Foundation and in part by NIH grant GM28016 to Marcus W. Feldman.
The expressions for the short- and long-term effect ( equation 10 in main text) can be reformulated as a function of LDs and allele frequencies. First, a short calculation shows that
where the terms s 1 and s 2 correspond to the selection on the interaction loci 1 and 2, respectively. Furthermore, the conditional allele frequencies ( p i m , p i M ) and LDs (LD M , LD m ) can be expressed in terms of allele frequencies ( p 1 , p 2 , p M ), two-way LDs (D 12 , D 1M , D 2M ) and three-way LD (D 12M ) ( Barton 1995 ; Shpak & Gavrilets 2006 ). With these transformations, the long- and short-term effects read
Following Barton (1995) , an exact expression for the long and short-term effects can be derived as follows. The total selection acting on the modifier, s M (see equation 8 in the main text) can be expressed in terms of allele-frequencies and LDs:
Thus, s M is the sum of the long-term effect which results from the association of the modifier with individual alleles ( D 1M and D 2M ) and the short-term effect which results from the association of the modifier with allele combinations ( D 12M ). Comparing this exact derivation of the long-term and short-term effects with the corresponding terms of the linear approximation ( A1 and A2 ), we see that the exact calculation and the linear approximation of the long-term effect are identical, while the exact calculation and the linear approximation of the short-term effect differ by a term proportional to D 1M D 2M . Given the properties of the linear approximation, this term is negligible for weak modifiers (a result we confirmed by numerical simulation).
- Agrawal AF, Otto SP. Host-parasite coevolution and selection on sex through the effects of segregation. Am. Nat. 2006; 168 :617–679. [ PubMed ] [ Google Scholar ]
- Barton NH. A General-Model for the Evolution of Recombination. Genet Res. 1995; 65 :123–144. [ PubMed ] [ Google Scholar ]
- Bell G. The Masterpiece of Nature: The Evolution and Genetics of Sexuality. University of California Press; 1982. [ Google Scholar ]
- Charlesworth B. Recombination Modification in a Fluctuating Environment. Genetics. 1976; 83 :181–195. [ PMC free article ] [ PubMed ] [ Google Scholar ]
- Gandon S, Otto SP. The evolution of sex and recombination in response to abiotic or coevolutionary fluctuations in epistasis. Genetics. 2007; 175 :1835–1853. [ PMC free article ] [ PubMed ] [ Google Scholar ]
- Haldane JBS. Disease and Evolution. La Ricerca Scientifica. 1949; 19 :2–11. [ Google Scholar ]
- Hill WG, Robertson A. The effect of linkage on the limits to artificial selection. Genetical Research, Cambridge. 1966; 8 :269–294. [ PubMed ] [ Google Scholar ]
- Kouyos RD, Salathé M, Bonhoeffer S. The Red Queen and the persistence of linkage-disequilibrium oscillations in finite and infinite populations. BMC Evolutionary Biology. 2007; 7 :211. [ PMC free article ] [ PubMed ] [ Google Scholar ]
- Kouyos RD, Salathé M, Otto SP, Bonhoeffer S. The role of epistasis on the evolution of recombination in host-parasite coevolution. Theoretical Population Biology. 2008 (2008), doi:10.1016/j.tpb.2008.09.007. [ PubMed ] [ Google Scholar ]
- Kover PX, Caicedo AL. The genetic architecture of disease resistance in plants and the maintenance of recombination by parasites. Mol. Ecol. 2001; 10 :1–16. [ PubMed ] [ Google Scholar ]
- Muller HJ. Some genetic aspects of sex. Am Nat. 1932; 66 :118–138. [ Google Scholar ]
- Nee S. Antagonistic Co-Evolution and the Evolution of Genotypic Randomization. J Theor Biol. 1989; 140 :499–518. [ PubMed ] [ Google Scholar ]
- Otto SP, Lenormand T. Resolving the paradox of sex and recombination. Nature Reviews Genetics. 2002; 3 :252–261. [ PubMed ] [ Google Scholar ]
- Otto SP, Nuismer SL. Species interactions and the evolution of sex. Science. 2004; 304 :1018–1020. [ PubMed ] [ Google Scholar ]
- Peters AD, Lively CM. The red queen and fluctuating epistasis: A population genetic analysis of antagonistic coevolution. Am Nat. 1999; 154 :393–405. [ PubMed ] [ Google Scholar ]
- Peters AD, Lively CM. Short- and long-term benefits and detriments to recombination under antagonistic coevolution. J Evolution Biol. 2007; 20 :1206–1217. [ PubMed ] [ Google Scholar ]
- Salathé M, Kouyous RD, Bonhoeffer S. The state of affairs in the kingdom of the Red Queen. Trends in Ecology and Evolution. 2008a; 23 (8):439–445. [ PubMed ] [ Google Scholar ]
- Salathé M, Kouyous RD, Regoes RR, Bonhoeffer S. Rapid Parasite Adaptation drives Selection for High Recombination Rates. Evolution. 2008b; 62 :295–300. [ PubMed ] [ Google Scholar ]
- Schmid-Hempel P, Jokela J. Socially structured populations and evolution of recombination under antagonistic coevolution. Am Nat. 2002; 160 :403–408. [ PubMed ] [ Google Scholar ]
- Shpak M, Gavrilets S. Population genetics: multilocus. Nature Encyclopedia of Life Sciences. 2006 [ Google Scholar ]
- Sturtevant AH, Mather E. The interrelations of inversions, heterosis and recombination. Am Nat. 1938; 72 :447–452. [ Google Scholar ]
- Wright S. The roles of mutation, inbreeding, crossbreeding and selection in evolution. VI International Congress of Genetics; 1932. pp. 356–366. [ Google Scholar ]
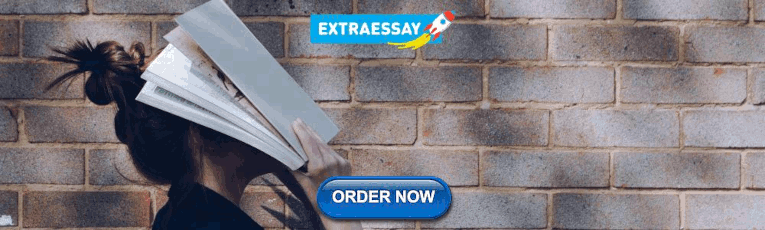
IMAGES
VIDEO
COMMENTS
Describe oscillating dynamics of the Red Queen hypothesis (don't have to be very specific) Diversity is maintained when rare genotypes or species become abundant and common genotypes or species become rarer. This process is caused by cyclical rises and falls in genotype frequency of matching hosts and pathogens.
the Red Queen hypothesis describes the reciprocal nature of host-parasite interactions, and that parasites are targeting the most common genotype, therefore parasites select for sexual reproduction reflecting Matching Allele model. In Agrawal and Lively (2002) they discuss differences between infection matrices.
Start studying red queen hypothesis. Learn vocabulary, terms, and more with flashcards, games, and other study tools.
The Red Queen's hypothesis is a hypothesis in evolutionary biology proposed in 1973, that species must constantly adapt, evolve, and proliferate in order to survive while pitted against ever-evolving opposing species.The hypothesis was intended to explain the constant (age-independent) extinction probability as observed in the paleontological record caused by co-evolution between competing ...
7.5 Testing the Red Queen Hypothesis. The Red Queen hypothesis—that sex evolved to combat our coevolving pathogens—can be tested by analyzing a few key predictions of this hypothesis: Sex is most beneficial where there is a high risk of infection. Pathogens are more likely to attack common phenotypes (for example, clones) in a population ...
The "Red Queen" hypothesis in evolution is related to the coevolution of species. It states that species must continuously adapt and evolve to pass on genes to the next generation and also to keep from going extinct when other species within a symbiotic relationship are evolving. First proposed in 1973 by Leigh Van Valen, this part of the ...
41. Testing the Red Queen Hypothesis. The Red Queen hypothesis—that sex evolved to combat our coevolving pathogens—can be tested by analyzing a few key predictions of this hypothesis: Sex is most beneficial where there is a high risk of infection. Pathogens are more likely to attack common phenotypes (for example, clones) in a population ...
Red Queen hypothesis. The idea that, in order for a species to maintain a particular niche in an ecosystem and its fitness relative to other species, that species must be constantly undergoing adaptive evolution because the organisms with which it is coevolving are themselves undergoing adaptive evolution. When species evolve in accordance with ...
Leigh Van Valen's famous Red Queen hypothesis is firmly established in evolutionary biology textbooks. It states that species accumulate small changes to keep up with a continually changing ...
The Red Queen Effect or Red Queen Hypothesis is a term coined by Leigh Van Valen to explain the ever-changing nature of evolution by natural selection. The hypothesis states that the likelihood of extinction for any given species remains relatively constant over time. Although a species in a given environment may have an advantage at one point ...
The Red Queen hypothesis was proposed over 40 years ago by the late evolutionary biologist Leigh Van Valen. It advanced evolutionary thinking beyond the idea that organisms were merely matched to their physical environment by suggesting that interactions between species (such as between hosts and parasites, predators and prey) would also be ...
Search for: 'Red Queen hypothesis' in Oxford Reference ». A hypothesis, proposed by L. M. Van Valen in the early 1970s, that describes how the coevolution of competing species creates a dynamic equilibrium, in which the probability of extinction remains fairly constant over time. Hence, evolution is seen neither as 'progressive' - with a ...
The Red Queen Hypothesis and it's Relevance. The statement that sparked this hypothesis is "Now, here, you see, it takes all the running you can do, to keep in the same place" (Carroll 1871 ). Van Valen's reference is essentially a metaphor for an evolutionary arms race. Predators that undergo a beneficial adaption may spark a change in ...
The Red Queen hypothesis rests on the idea that species must continuously evolve just to hang on to their ecological niche. That gradual evolution is driven by the constant genetic churn of sexual ...
Biologist Robert Vrijenhoek has been studying the Mexican poeciliid fish for more than 30 years. Some species of Poeciliopsis reproduce sexually while others reproduce asexually. Vrijenhoek found that the genetic diversity produced by sexual reproduction allowed the sexual fish to survive a parasite more successfully than the asexual fish.
Van Valen's 'Red Queen hypothesis' (RQH) emphasized the primacy of biotic interactions over abiotic forces in driving evolution. This was a revolutionary advance in biological thinking on the sources and modes of selection driving evolutionary change. Previously, the view of evolution by natural selection was that of a 'hill climbing ...
Abstract. Viruses and hosts must navigate environments in which each tries to outcompete the other for survival or to coexist within the same spaces. In Lewis Carrol's Through the Looking Glass, the Red Queen tells Alice, "Now, here, you see, it takes all the running you can do, to keep in the same place. If you want to get somewhere else, you ...
The Red Queen hypothesis (RQH) is both familiar and murky, with a scope and range that has broadened beyond its original focus. Although originally developed in the palaeontological arena, it now encompasses many evolutionary theories that champion biotic interactions as significant mechanisms for evolutionary change. As such it de-emphasizes ...
The Red Queen hypothesis. The assumption that fitness landscapes are constant over time is overly simplistic for many biological scenarios. Thus an alternative hypothesis to explain the ubiquity of genetic recombination is that it may continually create novel genotypes that are at a selective advantage in an ever-changing environment.