Disclaimer » Advertising
- HealthyChildren.org
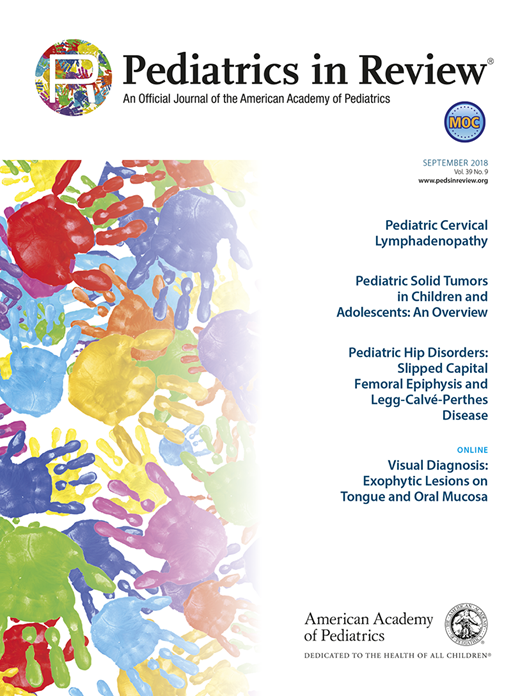
- Previous Article
- Next Article
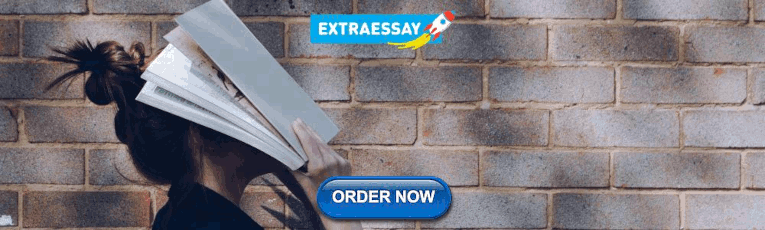
Presentation
The condition, management and prognosis, patient course, lessons for the clinician, case 1: recurrent pneumonia in a 15-year-old girl.
AUTHOR DISCLOSURE
Drs Jichlinski , Kilaikode, and Koumbourlis have disclosed no financial relationships relevant to this article. This commentary does not contain a discussion of an unapproved/investigative use of a commercial product/device.
- Split-Screen
- Article contents
- Figures & tables
- Supplementary Data
- Peer Review
- CME Quiz Close Quiz
- Open the PDF for in another window
- Get Permissions
- Cite Icon Cite
- Search Site
Amanda Jichlinski , Sasikumar Kilaikode , Anastassios C. Koumbourlis; Case 1: Recurrent Pneumonia in a 15-year-old Girl. Pediatr Rev September 2018; 39 (9): 464–467. https://doi.org/10.1542/pir.2017-0205
Download citation file:
- Ris (Zotero)
- Reference Manager
A previously healthy 15-year-old girl presents with a history of back pain, chills, and shortness of breath of 1 day’s duration. On examination she is afebrile and well appearing despite mild tachypnea (respiratory rate of 24 breaths/min). Oxyhemoglobin saturation is normal (95%–100%) on room air. On auscultation she has absent sounds in the left lower lung (LLL) fields but normal breath sounds on the right. Initial laboratory tests show a white blood cell count of 13,050/μL (13.05 × 10 9 /L), a hemoglobin level of 14.1 g/dL (141 g/L), a hematocrit value of 40.5%, and a platelet count of 226 × 10 3 /μL (226 × 10 9 /L). A basic metabolic panel is normal, and lactate dehydrogenase and uric acid levels are within their respective reference ranges. The C-reactive protein level is elevated at 8.56 mg/L (81.53 nmol/L). A chest radiograph (CXR) reveals a large area of consolidative opacity in the left hemithorax (compatible with pneumonia and/or atelectasis) and probable pleural effusion ( Fig 1 ).
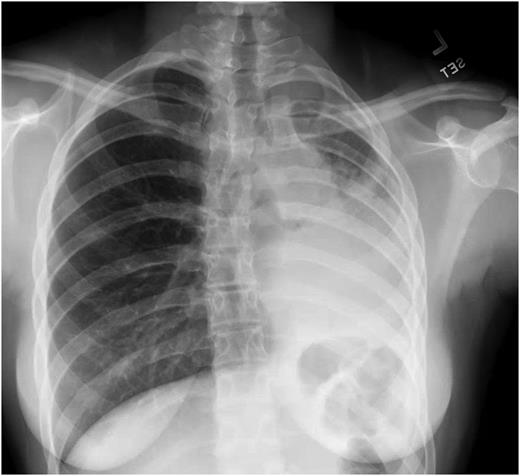
Chest radiograph on hospital admission.
Her medical history is notable for a diagnosis of mild asthma and an episode of pneumonia 18 months before presentation, diagnosed clinically without CXR and treated with antibiotics as an outpatient. She was also admitted to the hospital twice with a diagnosis of LLL pneumonia and pleural effusion 7 and 6 months before this admission. She responded promptly to antibiotic therapy, and no other intervention was taken. At a follow-up appointment after the second hospitalization she was well appearing but had decreased breath sounds on the left base. Her pulmonary function test result was consistent with mild to moderate restrictive lung defect. A CXR revealed a residual density in the LLL that was thought to represent the gradual clearing of the previous pneumonia.
The patient is admitted to the hospital for further evaluation and treatment with intravenous antibiotics. Further imaging reveals the diagnosis.
Recurrent pneumonia in children is always a cause of concern because it often results from underlying conditions or anatomical abnormalities that can be summarized as follows: 1) immunodeficiencies (genetic or acquired), 2) conditions that promote the accumulation of mucus into the lungs (eg, poorly controlled asthma or cystic fibrosis), 3) conditions that impair the clearance of mucus from the airways (eg, primary ciliary dyskinesia or tracheobronchomalacia), 4) structural abnormalities of the lungs (eg, cystic pulmonary airway malformations), and 5) indolent infections (eg, tuberculosis).
The patient’s CXRs all showed partial consolidation of the LLL that had improved during her follow-up appointment. Recurrent pneumonia in the same location is usually associated with an anatomical abnormality and raises suspicion for pulmonary sequestration.
Although the patient was admitted to the hospital 3 times with the diagnosis of pneumonia with pleural effusion, there were elements of her presentation that made a primary diagnosis of infectious pneumonia less likely. Specifically, she did not have high fever and leukocytosis, and despite the impressive consolidation on CXR she was not hypoxemic, suggesting that there was no acute, severe ventilation/perfusion mismatching.
Chest ultrasonography showed only a small pleural effusion and evidence of collapse versus consolidation of most of the left lung. A computed tomographic (CT) scan of the chest revealed a mass lesion at the origin of the left main bronchus, consolidation and collapse of the left lung, a small circumferential pleural effusion, and marked leftward mediastinal shift ( Fig 2 ).
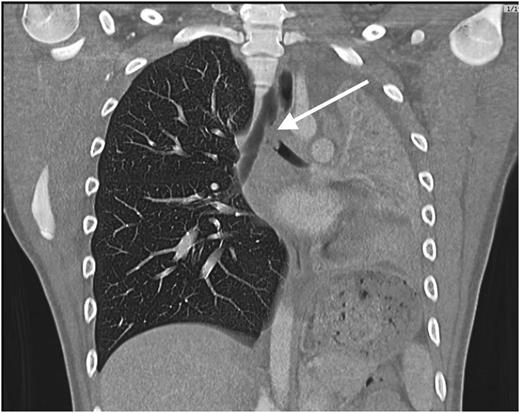
Computed tomographic scan of the chest revealing a filling defect in the left mainstem bronchus, collapse of the left lung, and compensatory hyperinflation of the right lung.
Flexible bronchoscopy revealed a large, irregular, lobulated, “cauliflower-like” mass at the lower left wall of the trachea that was obstructing the takeoff of the left mainstem bronchus and part of the carina. The mass was soft and fleshy ( Fig 3 ). The right mainstem bronchus and its lobar and segmental bronchii were patent. Multiple forcep biopsies were obtained. Pathologic evaluation revealed large cells with abundant cytoplasm that stained positive for vimentin and S100, consistent with a benign granular cell tumor. Bronchial washings were obtained and were negative for bacterial, fungal, or viral pathogens. The tuberculin purified protein derivative test result was negative.
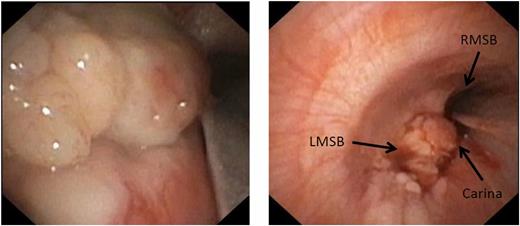
Bronchoscopic appearance of the tumor obstructing the takeoff of the left mainstem bronchus (LMSB), the open right mainstem bronchus (RMSB), and the carina.
Granular cell tumors (GCTs) originate from Schwann cells, although the exact pathology leading to their formation is unknown. ( 1 ) Lung GCTs are extremely rare, accounting for less than 10% of all GCTs, the incidence of which has been estimated to be 5 cases per million person-years in the general population. The incidence in the pediatric population is significantly lower. ( 2 )( 3 ) Pulmonary GCTs tend to be found in the larger airways at bifurcation sites, with a higher number of reported pediatric cases originating in the larynx. ( 4 ) Although most often benign, several cases of malignant GCTs have been reported in adults. ( 5 )
Pathologically, GCTs are composed of polygonal or ovoid cells with large eosinophilic, granular cytoplasms. The nuclei are often small, hyperchromatic, and eccentric, with absent mitosis. S-100 protein, neuron-specific enolase, CD56, and chromogranin are expressed in pulmonary GCTs, consistent with the pathology reported in the present case. ( 6 )
The differential diagnosis for bronchial and tracheal masses is large and includes pulmonary carcinomas, hemangiomas, inflammatory pseudotumors, rhabdomyosarcomas, neurofibromas, and squamous cell carcinomas. ( 1 )( 7 ) Note that pneumonia and/or atelectasis may be the presenting symptom of an endobronchial lesion because the intraluminal mass prevents the clearance of secretions from the airways, thus predisposing the patient to infection. The fact that patients clinically improve with antibiotic therapy often delays diagnosis of the tumor.
Management of GCTs is still debated because few cases have been reported. The tumors do not respond to chemotherapy or radiotherapy, so surgical resection is always necessary. ( 6 ) The size of tumors and invasion of the tracheobronchial wall play a role in guiding surgical management; larger, more invasive tumors require complete resection of the lung via thoracic surgery. ( 8 )( 9 )
Some authors have advocated for endoscopic removal of smaller intraluminal lesions with close follow-up and monitoring for recurrence. ( 1 )( 10 )( 11 ) Although endoscopy may not always allow the complete resection of the tumor, it is less invasive than pneumonectomy and has significantly lower morbidity. Most importantly, there has never been documented malignancy of a GCT in the pediatric age group, and recurrence due to incomplete resection is rare (0%–12%). ( 1 )( 5 ) However, it must be kept in mind that given the paucity of cases, true recurrence rates remain unknown.
The patient’s tachypnea and back pain improved with intravenous antibiotic therapy, and she was discharged on oral antibiotics. The tumor was excised a few weeks later by endoscopic sleeve resection, obviating the need for lobar or total lung resection. The lung fully reexpanded postoperatively.
Our patient’s presentation illustrates the need to thoroughly investigate any recurrent or persistent “pneumonia.” Because no CT scan or bronchoscopy was performed during the first 2 hospital admissions, we cannot be absolutely certain that the tumor was present at the first admission. However, the lack of hypoxemia in the setting of absent breath sounds in the LLL field and severe atelectasis on CXRs suggest a slowly growing tumor that was gradually obstructing the LLL, allowing the diversion of the blood flow to the right lung and avoiding the development of ventilation/perfusion mismatching. It is likely that there was also a superimposed infectious process present because the patient clinically improved with the administration of antibiotics during all her hospital admissions. Superimposed bacterial infections are common in endobronchial lesions that impair the clearance of secretions and predispose the affected lung to colonization with bacterial organisms that may cause bacterial bronchitis and/or pneumonia.
Uncomplicated community-acquired pneumonia can be diagnosed and treated on the basis of the clinical presentation and physical findings alone, without the need for CXR. However, when the patient has recurrent episodes, it is virtually impossible to determine whether it is a recurrence of the same pneumonia or a new and unrelated episode. In addition, the radiographic findings of pneumonia may persist for weeks or even months after a single episode. Thus, it is imperative for primary care physicians to follow patients closely and monitor until there is complete clinical resolution. The diagnosis of 2 or more episodes of pneumonia should include CXR and a follow-up CXR several weeks after the clinical recovery to document resolution.
Recurrent or persistent “pneumonia” requires further investigation. A CT scan of the chest with contrast is a reasonable starting point. Suspected pleural effusions should be evaluated with ultrasonography to detect and quantify the presence of free fluid or loculations in the pleural cavity. Flexible bronchoscopy should be performed when an obstructing lesion is suspected and/or if bronchoalveolar lavage is needed to identify pathogens.
Recurrent pneumonia, especially in the same location, should raise suspicion for an underlying condition predisposing the patient to the pneumonia, and it should be thoroughly investigated.
Radiographic findings that are disproportionately severe relative to the clinical presentation suggest a chronic, slowly evolving process as opposed to an acute process that is usually associated with substantial distress and hypoxemia.
Flexible bronchoscopy should be considered when airway obstruction (either from an intraluminal mass or due to external compression or malacia) is suspected.
Parapneumonic effusions may be difficult to distinguish from atelectasis/consolidation if there is no free-flowing fluid that would layer in a decubitus position. Thus, their presence should be confirmed by chest ultrasonography and/or computed tomography with contrast.
Note . This case is based on a poster presentation by Drs Kilaikode, Jichlinski, and Koumbourlis at the American Thoracic Society International Conference; Washington, DC; May 22, 2017. Poster No. 8842.
EDITOR’S NOTE
We invite readers to contribute Index of Suspicion cases through the PIR manuscript submission system at: https://mc.manuscriptcentral.com/pir .
Competing Interests
Advertising Disclaimer »
Citing articles via
Email alerts.
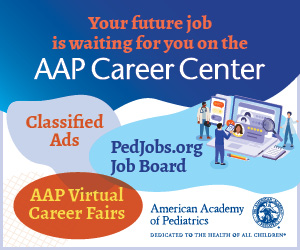
Affiliations
- Editorial Board
- ABP Content Spec Map
- Pediatrics On Call
- Online ISSN 1526-3347
- Print ISSN 0191-9601
- Pediatrics Open Science
- Hospital Pediatrics
- Pediatrics in Review
- AAP Grand Rounds
- Latest News
- Pediatric Care Online
- Red Book Online
- Pediatric Patient Education
- AAP Toolkits
- AAP Pediatric Coding Newsletter
First 1,000 Days Knowledge Center
Institutions/librarians, group practices, licensing/permissions, integrations, advertising.
- Privacy Statement | Accessibility Statement | Terms of Use | Support Center | Contact Us
- © Copyright American Academy of Pediatrics
This Feature Is Available To Subscribers Only
Sign In or Create an Account
- Research article
- Open access
- Published: 21 July 2008
Clinical case review: A method to improve identification of true clinical and radiographic pneumonia in children meeting the World Health Organization definition for pneumonia
- Taneli Puumalainen 1 ,
- Beatriz Quiambao 2 ,
- Erma Abucejo-Ladesma 2 ,
- Socorro Lupisan 2 ,
- Tarja Heiskanen-Kosma 1 ,
- Petri Ruutu 1 ,
- Marilla G Lucero 2 ,
- Hanna Nohynek 1 ,
- Eric AF Simoes 3 ,
- Ian Riley 4 &
the ARIVAC Research Consortium
BMC Infectious Diseases volume 8 , Article number: 95 ( 2008 ) Cite this article
11k Accesses
42 Citations
Metrics details
The World Health Organization's (WHO) case definition for childhood pneumonia, composed of simple clinical signs of cough, difficult breathing and fast breathing, is widely used in resource poor settings to guide management of acute respiratory infections. The definition is also commonly used as an entry criteria or endpoint in different intervention and disease burden studies.
A group of paediatricians conducted a retrospective review of clinical and laboratory data including C-reactive protein concentration and chest radiograph findings among Filipino children hospitalised in the Bohol Regional Hospital who were enrolled in a pneumococcal vaccine efficacy study and had an episode of respiratory disease fulfilling the WHO case definition for clinical pneumonia. Our aim was to evaluate which disease entities the WHO definition actually captures and what is the probable aetiology of respiratory infections among these episodes diagnosed in this population.
Among the 12,194 children enrolled to the vaccine study we recorded 1,195 disease episodes leading to hospitalisation which fulfilled the WHO criteria for pneumonia. In total, 34% of these episodes showed radiographic evidence of pneumonia and 11% were classified as definitive or probable bacterial pneumonia. Over 95% of episodes of WHO-defined severe pneumonia (with chest indrawing) had an acute lower respiratory infection as final diagnosis whereas 34% of those with non-severe clinical pneumonia had gastroenteritis or other non-respiratory infection as main cause of hospitalisation.
The WHO definition for severe pneumonia shows high specificity for acute lower respiratory infection and provides a tool to compare the total burden of lower respiratory infections in different settings.
Trial registration
ISRCTN62323832
Peer Review reports
The World Health Organization (WHO) has developed standard case management guidelines to reduce the two million deaths, or 20 percent of all child deaths, caused by pneumonia through early diagnosis and treatment [ 1 ]. A meta-analysis showed that these guidelines, if effectively implemented, result in significant reduction in mortality: 24% (95% CI 14–33%) in total mortality and 36% (95% CI 20–48%) in pneumonia-related mortality in children aged 0 to 4 years in developing countries with infant mortality over 90 per 1,000 live births [ 2 ]. Many countries have now adapted these guidelines as part of national acute respiratory infections control and Integrated Management of Childhood Illnesses (IMCI) programs.
The WHO guidelines define pneumonia as an acute disease episode with cough or difficult breathing combined with fast breathing with age specific cut-off values for increased respiratory rate. Children with lower chest wall indrawing classified as severe pneumonia are referred for evaluation and possible in-patient care. While it is estimated that these criteria detect over 80 percent of children that require antibiotic treatment for probable bacterial pneumonia or hospital care for severe disease, 20 to 30 percent of children fulfilling the criteria receive unnecessary antimicrobials for non-severe viral respiratory infection [ 3 ]. This is especially true for children with expiratory wheezing due to asthma, bronchiolitis or other viral respiratory infections who are often misclassified as pneumonia requiring antimicrobial treatment and referred for in-patient care [ 4 ].
Another problem of the low specificity of the WHO definition for pneumonia is its use as study enrolment criteria or one of the study endpoints in disease burden and intervention studies. For example, studies evaluating the efficacy of new conjugated vaccines against Streptococcus pneumoniae show these vaccines are effective in reducing invasive pneumococcal disease and radiographic pneumonia, but the efficacy is low for the WHO-defined clinical pneumonia [ 5 , 6 ].
We carried out a randomised, double-blinded, placebo-controlled study to evaluate the efficacy of an investigational 11-valent pneumococcal conjugate vaccine against childhood pneumonia in the Philippines. We used the WHO definition for pneumonia as entry criteria for clinical data collection from study subjects hospitalised and as a secondary endpoint for vaccine efficacy analysis. This report describes, without un-blinding the study randomisation code, results of a retrospective review of clinical, laboratory and radiological data from study subjects hospitalised with an illness that on admission fulfilled the WHO definition for pneumonia. Our aim was to describe the clinical methods used in our vaccine study and evaluate the use of WHO definition for clinical pneumonia in a South East Asian paediatric hospital setting. We attempted to improve the specificity of diagnosis of WHO defined clinical pneumonia by classifying the disease episodes into International Classification of Diseases, version 10 (ICD-10)-classification system categories for pneumonia which then could be used to analyse the vaccine efficacy. The results are discussed in light of possible different diagnostic approaches for respiratory infections, which could be utilised in pneumococcal or other vaccine and disease burden studies.
Study setting
The study was conducted in six municipalities in Bohol Province in central Philippines. At the beginning of the vaccine study in 2000, the predominantly agricultural area covering 357 Km 2 had a total population of 149,000. There is no malaria and practically no HIV in Bohol [ 7 ]. In 2005, the Gross National Income of the Philippines was 1,300 USD/capita. The infant mortality rate (IMR) in Bohol during years 1999–2002 was 28/1,000 live births, with the major causes of child deaths being pneumonia and diarrhoea [ 7 ].
Study subjects
This study to evaluate the efficacy of an 11-valent mixed carrier tetanus protein or diphtheria toxoid conjugated pneumococcal vaccine against radiographic pneumonia in children aged 6 weeks to 23 months started in July 2000. All children born in the study area and healthy enough to receive their first diphtheria, tetanus, whole-cell pertussis vaccine (DTwP) dose according to the national Expanded Program on Immunization (EPI) program were offered study participation. The vaccines administered included Bacillus-Calmette-Guerin (BCG) at 2 weeks of age, 3 doses of DTwP and Haemophilus influenzae type b (DTwP//PRP-T) conjugate vaccine, plasma derived hepatitis B vaccine and study vaccine or placebo (normal saline) starting at age of 6 weeks to < 6 months with a minimum interval of 4 weeks between the doses. Measles vaccine was given at 9 months of age.
Case ascertainment for pneumonia
Patients described in this paper were evaluated at the Bohol Regional Hospital, which is a 250-bed tertiary government hospital managing approximately 85% of children with severe infections aged less than 5 years from the study area [ 8 ]. Most of the children were walk-in patients without referral from the primary health care clinics. All children were seen within 30 minutes of arrival by a study nurse who screened whether the patient's age (6 weeks to 23 months), residency (six study municipalities) and symptoms met the criteria for data collection. The eligibility was then immediately reconfirmed by a physician. A community acquired pneumonia was defined as pneumonia with onset prior to or less than 72 hours following admission to hospital. The WHO criteria for non-severe pneumonia included: a history of cough and/or difficult breathing of less than 3 weeks duration, with (a) increased respiratory rate (Rate ≥ 60/min if age <2 months, ≥ 50/min if age 2–11 months and ≥ 40/min if age 12–59 months); (b) lower chest wall indrawing (severe pneumonia); or (c) cyanosis and/or inability to feed or drink (very severe pneumonia). In case of expiratory wheezing on auscultation the child was first given three doses of an inhaled bronchodilator, and the eligibility was re-assessed 30 minutes after the last dose. The child was further evaluated according to the study procedures if the signs and symptoms of WHO-defined pneumonia were still present after the bronchodilator treatment. New admission due to a disease meeting the WHO pneumonia criteria within 14 days of the previous evaluation was considered to belong to the same disease episode. The haemoglobin oxygen saturation at room air was measured with BCI 3303 oximeter (Waukesha, USA) after stabilization of the reading. A blood sample was drawn for bacterial culture, white blood cell count (Advia 60 automatic analyzer, Bayer, USA), and serum. The bacterial cultures were conducted by using standard methods [Methods described in detail in references [ 9 ] and [ 10 ]]. Regular quality control of bacterial culture techniques and findings were conducted by the Research Institute for Tropical Medicine in the Philippines and National Public Health Institute in Finland. Coagulase negative staphylococci, Bacillus, diphtheroids and gram negative non-fermenting organisms were considered contaminants. The serum samples were transported frozen to Finland for analysis of C-reactive protein by immunoturbidometry and radioimmunoassay (Hitachi Modular P1-analyser, Hitachi Ltd, Tokyo, Japan) at the Helsinki University Central Hospital. All patients were subjected to chest radiograph which was interpreted by using a reading process developed by the WHO [ 11 ]. The radiographs were also interpreted separately using a structured questionnaire by an independent pediatric radiologist blinded to the clinical data. Survival of all study subjects was checked by the study team at the age of 23 months, or following the termination of the study data collection in December 2004.
Quality assurance of case ascertainment
The study nurses and physicians were trained in the WHO pneumonia-management algorithm [ 12 , 13 ] and were monitored weekly for accuracy and consistency of measurement of respiratory rate, chest indrawing and other signs of pneumonia. The intensive monitoring kept the inter-observer variation in pneumonia severity assessment at less than 10% during the study period. All data were double entered into the study database. An independent monitoring team consisting of clinical research associates constantly checked the integrity of all clinical study data.
Review of patient data by the clinical review team
The retrospective review of clinical data collected during 2000 to 2004 from each disease episode was carried out from November 2003 to March 2005 by a team consisting paediatricians familiar with the study setting (TP, BQ, EA-L, SL, TH-K). Structured data from the study database (as shown in Table 1) and non-structured admission and daily follow-up data from the hospital records, as well as results of laboratory and radiology investigations were used as source data. The review team utilised the International Classification of Diseases, version 10 (ICD-10) coding system to record final diagnoses for each disease episode. Disease episodes with clinical signs and symptoms of infection and infiltrates or pleural fluid in chest radiograph were classified as pneumonia whereas those episodes with normal or missing chest radiograph as clinical lower respiratory tract infection. The review team also further classified the pneumonia episodes according to the probable aetiology, i.e. to bacterial, mixed bacterial-viral and viral infections, by using the best clinical judgement based on clinical data, C-reactive protein concentration, white blood cell count, radiographic appearance and response to treatment with or without antimicrobials. Underlying medical conditions such as malnutrition and congenital abnormalities were also registered. The most important medical condition leading to hospitalisation was regarded as the primary diagnosis. The intra-observer variability of the review process was evaluated in approximately 10 percent of episodes by repeating the review several months later blinded to the results of the first review.
Ethical review and approvals
The parents or guardians of study subjects signed an informed consent before enrolment to the vaccine study. All therapeutic decisions were done independently by regular hospital physicians not belonging to the study team. The study was conducted in accordance with the latest South African revision of the Declaration of Helsinki, ICH Good Clinical Practice, and local regulatory requirements.
The concept of the trial submitted under title "Phase III Trial on the Efficacy of an 11-Valent Pneumococcal Conjugate Vaccine in the Reduction of Severe and Very Severe Pneumonia Among Filipino children Under 2 Years of Age" was approved by the Ethical and Institutional Review Board of the Research Institute of Tropical Medicine, the Philippines in November 1998. The final version of the trial protocol was approved in June 2000.
Statistical analysis
This report describes the clinical picture of pneumonia requiring hospitalisation and the method of clinical review used in our vaccine efficacy study context. No statistical hypothesis testing was conducted. We calculated Kappa coefficient for the reproducibility of the review.
The planned cohort of 12,194 infants was enrolled the vaccine efficacy study by December 2003 (78% of the total birth cohort, 92% of the non-transient population eligible for the study). Overall, 98.7% of the enrolled subjects received 3 doses of study vaccine. The median age at vaccination was 1.8, 2.9 and 3.9 months for first, second and third dose, respectively. Vaccine and placebo groups did not differ in key baseline socio-economic indicators (data not shown). The data on all hospital admissions and other important medical events were collected until study termination visit at 23 months of age (N = 8,780) or end of study follow up at the end of December 2004 (N = 2,366). Altogether 64 children died and 984 children were lost to follow up due to migration or were withdrawn from the study for various reasons.
This analysis covers 1,195 disease episodes fulfilling the WHO criteria for pneumonia among 821 children (60% boys) who were hospitalised at the Bohol Regional Hospital. The age distribution is shown in Table 1 [see Additional file 1 ]. According to the WHO pneumonia severity classification algorithm, 290 episodes (24%) were non-severe pneumonia i.e. without chest indrawing or other danger signs, 785 (66%) were severe pneumonia presenting with chest indrawing and 120 (10%) were very severe pneumonia with either inability to drink or central cyanosis. A blood culture was obtained in 90% of episodes. We detected 13 (1.1% of episodes) invasive bacterial infections. The most common bacterial pathogens included Staphylococcus aureus, Streptococcus pneumoniae and Salmonella Typhi. Chest radiographs from 182 (15.2%) episodes showed abnormal findings which met the WHO criteria for probable bacterial pneumonia (Primary endpoint consolidation). The mean duration of hospitalisation was 3.2 days (Range 0 to 32 days). Altogether 19 patients died while in hospital or within 14 days from discharge (Case fatality rate 1.6%).
The distribution of key clinical, laboratory and radiology findings in each of the WHO pneumonia severity categories is shown in Table 1 [see Additional file 1 ]. In episodes of severe, but not very severe pneumonia, 51% of children presented with expiratory wheeze compared to 9.3% in the non-severe and 40.8% in the very severe pneumonia categories. Very severe pneumonia was associated with highest probability for abnormal radiograph and hypoxemia, as well as the highest case fatality rate: 9.2% compared to 0.3% in non-severe pneumonia and 0.5% in severe pneumonia categories.
The WHO classifications of severe and very severe pneumonia identified acute lower respiratory infection very well (95% and 85% respectively) whereas the non-severe pneumonia classification identified only 49% of subjects with ALRI. According to the retrospective review of clinical, laboratory and radiographic data, 402 episodes (33.6%) had radiographically confirmed pneumonia as the major cause of hospitalisation (Table 2) [see Additional file 1 ]. Bacteriologically confirmed bacterial pneumonia or probable bacterial pneumonia was the diagnosis in 8.6%, 11.5% and 15.9% of episodes in non-severe, severe and very severe WHO pneumonia categories, respectively. Viral lower respiratory infection i.e. viral pneumonia or bronchiolitis was most common in the severe pneumonia category: 37.5% compared to 14.5% and 25.9% in non-severe and very severe pneumonia categories, respectively.
A total of 203 episodes (17.0%) were evaluated as not having a lower respiratory infection as the main reason for hospitalisation. Non-respiratory diseases, of which acute gastroenteritis was the most common, accounted for 12.7% of hospitalisations. The other non-respiratory diagnoses included a broad range of paediatric diseases such as acute febrile infections with seizures, skin infections, urinary tract infections and 9 cases of central nervous system infections.
Episodes which fulfilled the WHO criteria for non-severe pneumonia had the highest likelihood for having a non-respiratory disease as the main diagnosis. However, in 15 of the 37 episodes of acute gasteroenteritis the child had also signs of lower respiratory infection (3 with radiographic abnormalities). In total, seven of the 290 (2.4%) of episodes were diagnosed as sepsis or meningitis. In the severe pneumonia group, other than lower respiratory infection diagnoses were rare. The 12 episodes of gasteroenteritis included one episode, in which the patient also had a diagnosis of viral pneumonia. The 11 other episodes presented with chest indrawing on admission, but this could have been due to dehydration or simultaneous lower respiratory infection which did not show any radiographic abnormalities. Only in two episodes, the final diagnosis was meningitis or sepsis without pneumonia. The other diagnoses in the very severe pneumonia group included five episodes of sepsis, four episodes of central nervous system infections and three episodes of acute gastroenteritis. One episode was classified as an upper respiratory infection.
In order to ensure the reproducibility of the review, 111 episodes (9.3%) were re-evaluated several months later by the same review team who were blinded to the results of the first review round. The team assigned the same ICD-10 coded diagnosis in 79 of the 111 episodes evaluated (71.2%). Additional 21 episodes (18%) had diagnosis in the same disease group, but the ICD-10 coding differed (e.g. J22 non-specified lower respiratory infection vs. J21.9 bronchiolitis). A total of 37 episodes were assigned a pneumonia group diagnosis in the first review compared to 44 in the second review (Kappa coefficient 0.79). According to both reviews 93 episodes had a diagnosis belonging to any of lower respiratory tract infection categories (Kappa 0.87).
Our study demonstrates that in this population of Filipino children the WHO definition of clinical pneumonia captures a broad spectrum of respiratory infections varying from mild upper respiratory infections to severe bacterial pneumonia. In total, one third of episodes showed radiographic evidence of pneumonia and one third of these (11.2% of the total) were classified by the group of paediatricians as definitive or probable bacterial pneumonia. The severity of the disease correlated well with the WHO pneumonia severity algorithm. The non-severe pneumonia group had a higher probability for upper respiratory and non-respiratory group diagnoses than the severe or very severe pneumonia groups which identified very well the lower respiratory and other severe infections.
The findings of this study are in conformity with other studies conducted in the Philippines [ 14 , 15 ] and in other Asian countries [ 4 ] reporting a similar clinical picture in patients with a WHO-defined pneumonia. The case fatality rate of 0.5% observed in the severe pneumonia group was similar to that in a recent multi-centre study evaluating antimicrobial treatment alternatives for WHO-defined severe pneumonia [ 16 ], but lower than the 2.1% and 12% mortality described in Bohol, the Philippines during 1990's and in Lombok, Indonesia among children hospitalised for acute lower respiratory infection, respectively [ 17 , 18 ]. This may reflect the different patterns in care seeking behaviour and access to care.
The microbial aetiology of respiratory infection is difficult to determine, as the sensitivity of tests, especially bacterial cultures, is low, and mixed bacterial and viral infections are common. The chest radiograph and non-specific laboratory markers such as C-reactive protein, procalcitonin and white blood cell count provide some guidance, but the results overlap significantly in viral and bacterial pneumonia [ 19 , 20 ]. In this study the WHO classification of non-severe, severe and very severe pneumonia appeared to correlate with disease severity, but poorly with the probability of definitive or probable bacterial aetiology for infection. The distributions of C-reactive protein concentration and blood leukocyte count did not suggest major differences in proportions of episodes with bacterial aetiology in the different severity categories of pneumonia. Despite special attention to laboratory techniques the ratio of positive blood culture findings was low in all pneumonia severity categories. This may be partly explained by Hib vaccination provided to all study participants and pre-hospital antimicrobial treatment, which was, according to a parental questionnaire, 35% in our previous epidemiologic study conducted in Bohol during 1995–99 [ 9 ]. Nevertheless the overall ratio of invasive pneumococcal infections is far lower than those seen in studies in Africa [ 21 ]. In this study 15.2% of episodes showed a primary endpoint consolidation in chest radiograph. The frequency of chest radiograph findings increased with the pneumonia severity. The Gambian pneumococcal study, which used similar WHO criteria for case ascertainment, reported a primary endpoint radiographic finding in 18.1% of hospitalized children [ 5 ]. However, in the Gambian study, 4.2% of hospitalized patients had a blood culture or CSF positive for Streptococcus pneumonia compared to 0.3% in this study population.
Other studies have reported 65% to 90% specificity of the WHO pneumonia criteria for lower respiratory infection [ 3 , 12 , 13 , 21 ]. In our study, the retrospective clinical review demonstrated that in 17% of episodes the child showed signs and symptoms of WHO-defined pneumonia, but the main condition prompting the decision to admit the patient for hospital care was not the acute lower respiratory infection. Largely this consists of patients with non-severe pneumonia, of which over half had another concomitant disease that was the main reason for hospitalisation. According to the WHO guidelines the children with non-severe pneumonia should not be referred for hospital care, but provided with oral antibiotics for acute respiratory infection. Among children with severe pneumonia presenting with chest indrawing, the frequency of concomitant disease prompting the decision for admission was low (< 5%). The episodes of very severe pneumonia included, in addition to lower respiratory infections, also other severe infections. This finding supports the current practise of including these patients to the IMCI guidelines category of severe disease needing urgent referral to hospital care.
We noted expiratory wheeze in half of episodes of WHO defined severe pneumonia. This finding emphasises the importance of including the management of wheeze to the current pneumonia management guidelines. It is not known whether all children with wheeze, presumable caused by a viral infection, require antimicrobial therapy and, if not, which additional clinical findings would support withholding antibiotics [ 3 , 4 ]. The WHO currently recommends that children without previous history of wheeze who develop a lower respiratory infection with wheeze and tachypnoea may have a bacterial or mixed bacterial-viral infection, and should always be treated with antibiotics for suspected bacterial pneumonia [ 23 ]. The findings from the South African pneumococcal study, which reported 31% efficacy of the vaccine against pneumonia associated to respiratory viruses support this strategy [ 24 ]. The situation may, however, be different in many Asian countries where the incidences of wheezing diseases and paediatric asthma have increased and mortality attributable to severe respiratory infections has decreased [ 3 , 25 , 26 ].
A limitation of this study is the uncertainty of the role of the pneumococcal conjugate vaccine in modifying the clinical picture of respiratory infections. Other pneumococcal vaccine efficacy studies suggest that the vaccine may prevent 20–36% of radiographic pneumonia [ 5 , 6 ]. It is thus possible that viral respiratory infections are enriched in this patient population. We assumed that in 5% of episodes where a chest radiograph was not obtained the clinical review diagnosis was not pneumonia. While probably missed some infiltrates we do not believe that the overall interpretation of the data would have changed significantly. The diagnostic procedures conducted in this study represent practices available in many hospitals in South East Asian countries. The results of this study are not, however, directly applicable to other health care settings, in which the clinical picture of WHO-defined clinical pneumonia may be different. The reproducibility of our clinical case review process was high in our own study settings, but it is difficult to know if this process, based primarily on clinicians' best judgement, could be repeated in similar manner elsewhere. Whether the classification according to probable aetiology of infection was successful can be evaluated once we analyse the vaccine efficacy in different ICD-10 categories of respiratory infections and complete the on-going testing of respiratory viruses from nasopharyngeal samples collected from hospitalized study children.
The WHO case management algorithm for pneumonia provides an important tool for managing respiratory infection in resource poor settings. It also provides a tool to compare the total burden of respiratory infections in different communities, but should not, without additional microbiological evidence, be used to compare the burden attributable to specific respiratory pathogens. This study suggests that the category of WHO-defined severe pneumonia is very specific for lower respiratory infections whereas the non-severe pneumonia category includes, at least in hospital settings, a large proportion of other diseases.
The WHO definition for clinical pneumonia captures a broad spectrum of different paediatric respiratory diseases. The WHO classification of non-severe, severe and very severe pneumonia correlated well with disease severity, but poorly with the probability of definitive or probable bacterial aetiology for infection. The category of severe pneumonia presenting with chest indrawing showed high specificity for lower respiratory infection, and could be used when comparing the burden of respiratory infections in resource poor countries.
Williams BG, Gouws E, Boschi-Pinto C, Bryce J, Dye C: Estimates of worldwide distribution of child deaths from acute respiratory infections. Lancet Infect Dis. 2002, 2: 25-32. 10.1016/S1473-3099(01)00170-0.
Article PubMed Google Scholar
Sazawal S, Black RE: Effect of pneumonia case management on mortality in neonates, infants, and preschool children: a meta-analysis of community-based trials. Lancet Infect Dis. 2003, 3: 547-556. 10.1016/S1473-3099(03)00737-0.
World Health Organization: Consultative meeting to review evidence and research priorities in the management of acute respiratory infections. WHO/ARI/04.2. 2003, Geneva: WHO
Google Scholar
Hazir T, Qazi S, Nisar YB, Ansari S, Maqbool S, Randhawa S, Kundi Z, Ashar R, Aslam S: Assessment and management of children aged 1–59 months presenting with wheeze, fast breathing, and/or lower chest indrawing; results of a multicentre descriptive study in Pakistan. Arch Dis Child. 2004, 89: 1049-54. 10.1136/adc.2003.035741.
Article CAS PubMed PubMed Central Google Scholar
Cutts FT, Zaman SMA, Enwere G, Jaffar S, Levine OS, Okoko JB, Oluwalana C, Vaughan A, Obaro SK, Leach A, McAdam KP, Biney E, Saaka M, Onwuchekwa U, Yallop F, Pierce NF, Greenwood BM, Adegbola RA, for the Gambian Pneumococcal Vaccine Trial Group: Efficacy of nine-valent pneumococcal conjugate vaccine against pneumonia and invasive pneumococcal disease in The Gambia: randomised, double-blind, placebo-controlled trial. Lancet. 2005, 365: 1139-46. 10.1016/S0140-6736(05)71876-6.
Article CAS PubMed Google Scholar
Klugman KP, Madhi SA, Huebner RE, Kohberger R, Mbelle N, Pierce N: A Trial of a 9-valent pneumococcal conjugate vaccine in children with and those without HIV infection. N Eng J Med. 2003, 349: 1341-8. 10.1056/NEJMoa035060.
Article CAS Google Scholar
Philippine Health Statistics: Department of Health National Epidemiology Center, Manila. 2000
Lupisan SP, Herva E, Nohynek H, Lucero MG, Sombrero LT, Quiambao BP, Abucejo EP, Arcay J, Mäkelä PH, Ruutu P: Incidence of invasive Haemophilus influenzae type b infections in Filipino children. Pediatr Infect Dis J. 2000, 19 (10): 1020-22. 10.1097/00006454-200010000-00020.
Lupisan SP, Herva E, Sombrero LT, Quiambao BP, Capeding MR, Abucejo EP, Esparar G, Arcay J, Ruutu P: Invasive bacterial infections of children in a rural province in the central Philippines. Am J Trop Med Hyg. 2000, 62 (3): 341-6.
CAS PubMed Google Scholar
Herva E, Sombrero L, Lupisan S, Arcay J, Ruutu P: Establishing a laboratory for surveillance of invasive bacterial infections in a tertiary care government hospital in a rural province in the Philippines. Am J Trop Med Hyg. 1999, 60: 1035-40.
World Health Organization Pneumonia Vaccine Trial Investigators' Group: Standardization of interpretation of chest radiographs for the diagnosis of pneumonia in children. WHO/V&B/01.35, Geneva. 2001
Cherian T, John TJ, Simoes E, Steinhoff MC, John M: Evaluation of simple clinical signs for the diagnosis of acute lower respiratory track infection. Lancet. 1988, 2 (8603): 125-8. 10.1016/S0140-6736(88)90683-6.
World Health Organization Programme for the Control of Acute Respiratory Infections: Acute respiratory infections in children: Case management in small hospitals in developing countries. WHO/ARI/90.5. Geneva. 1990
Tupasi TE, Velmonte MA, Sanvictores ME, Abraham L, De Leon LE, Tan SA, Miguel CA, Saniel MC: Determinants of morbidity and mortality due to acute respiratory infections: implications for intervention. J Infect Dis. 1988, 157: 615-23.
Tupasi TE, Lucero MG, Magdangal DM, Mangubat NV, Sunico ME, Torres CU, de Leon LE, Paladin JF, Baes L, Javato MC: Etiology of acute lower respiratory infection in children in Alabang, Metro Manila. Rev Infect Dis. 1990, 12 (suppl 8): S929-939.
Addo-Yobo E, Chisaka N, Hassan M, Hibberd P, Lozano JM, Jeena P, MacLeod WB, Maulen I, Patel A, Qazi S, Thea DM, Nguyen NT: Oral amoxicillin versus injectable penicillin for severe pneumonia in children aged 3 to 59 months: a randomised multicentre equivalency study. Lancet. 364 (9440): 1141-8. 10.1016/S0140-6736(04)17100-6. 2004 Sep 25-Oct 1
Lupisan SP, Ruutu P, Abucejo-Ladesma PE, Quiambao BP, Gozum L, Sombrero LT, Romano V, Riley I, Simoes EAF: Central nervous system infection is an important cause of death in underfives hospitased with World Health Organization (WHO) defined severe and very severe pneumonia. Vaccine. 2007, 25: 2437-2444. 10.1016/j.vaccine.2006.09.017.
Djelantik IG, Gessner BD, Sutanto A, Steinhoff M, Linehan M, Moulton LH, Arjoso S: Case fatality proportions and predictive factors for mortality among children hospitalized with severe pneumonia in a rural developing country setting. J Trop Pediatr. 2003, 49 (6): 327-32. 10.1093/tropej/49.6.327.
Simon L, Gauvin DK, Saint-Louis P, Lacroix J: Serum procalcitonin and C-reactive protein levels as markers of bacterial infection: a systematic review and meta-analysis. Clin Infect Dis. 2004, 39: 206-17. 10.1086/421997.
Virkki R, Juven T, Rikalainen H, Svedström E, Mertsola J, Ruuskanen O: Differentation of bacterial and viral pneumonia in children. Thorax. 2002, 57: 438-41. 10.1136/thorax.57.5.438.
Shann F: Etiology of pneumonia in children in developing countries. Pediatr Infect Dis J. 1986, 5: 247-52.
Madico G, Gilman R, Jabra A, Rojas L, Hernandez H, Fukuda J, Bern C, Steinhoff M: The role of pulse oximetry: its use as an indicator of severe respiratory disease in Peruvian children living at sea level. Arch Pediatr Adolesc Med. 1995, 149: 1259-63.
World Health Organization: Bronchodilators and other medications for the treatment of wheeze-associated illnesses in young children. Programme for the Control of Acute Respiratory Infections. [ http://www.who.int/child-adolescent-health/New_Publications/CHILD_HEALTH/WHO_ARI_93.29.htm ]
Madhi SA, Klugman KP, Vaccine trialists group: A role of Streptococcus pneumoniae in virus-associated pneumonia. Nat Med. 2004, 10 (8): 811-3. 10.1038/nm1077.
Hong SJ, Lee MS, Sohn MH, Shim JY, Han YS, Park KS, Ahn YM, Son BK, Lee HB, Korean ISAAC Study Group: Self-reported prevalence and risk factors of asthma among Korean adolescents: 5-year follow-up study, 1995–2000. Clin Exp Allergy. 2004, 34 (10): 1556-62. 10.1111/j.1365-2222.2004.02084.x.
The International Study of Asthma and Allergies in Childhood (ISAAC) Steering Committee: Worldwide variation in prevalence of symptoms of asthma allergic rhinoconjunctivitis, and atopic eczema: ISAAC. Lancet. 1998, 351 (9111): 1225-1232. 10.1016/S0140-6736(97)07302-9.
Article Google Scholar
Pre-publication history
The pre-publication history for this paper can be accessed here: http://www.biomedcentral.com/1471-2334/8/95/prepub
Download references
Acknowledgements
This study is part of the research of the ARIVAC Consortium. We are indebted to the Consortium study team and the following collaborators: Dr Thelma Laot, Dr Romel Besa, Lydia Sombrero MSc, Antti Nissinen PhD, Prof Gail Williams, Prof P. Helen Mäkelä and families in Bohol Province.
The ARIVAC consortium consists of the National Public Health Institute, Finland, the Research Institute for Tropical Medicine, the Philippines, University of Queensland, Australia, sanofi pasteur (formerly known as Aventis Pasteur), France, Imperial College, UK and University of Colorado, USA. Funding of the study was provided by all members of the ARIVAC consortium, and the European Commission DG Research INCO programme (contracts IC18-CY97-2019, ICA4-CT-1999-10008, ICA4-CT-2002-10062), Academy of Finland (contracts 206283, 106974, 108873 and 108878), Finnish Ministry of Foreign Affairs (bilateral contracts 75502901 and 327/412/2000), the Finnish Physicians for Social Responsibility, the Global Alliance for Vaccines and Immunization-ADIP Pneumo-program, PATH and WHO. The investigational vaccines as well as the concomitant vaccines were provided by sanofi pasteur. Of these, only the consortium members were active in all phases of the study: its design; the collection, analysis, and interpretation of data; writing of a paper, and deciding to submit it for publication. PATH reviewed a final draft before submission. The views expressed by the authors do not necessarily reflect the views of PATH. The WHO funded DSMB reviewed safety and other data and interacted with the consortium throughout the trial itself.
Author information
Authors and affiliations.
National Public Health Institute, Mannerheimintie 166, FIN-00300, Helsinki, Finland
Taneli Puumalainen, Tarja Heiskanen-Kosma, Petri Ruutu & Hanna Nohynek
Research Institute for Tropical Medicine, Metro Manila, Philippines
Beatriz Quiambao, Erma Abucejo-Ladesma, Socorro Lupisan & Marilla G Lucero
University of Colorado, Denver, USA
Eric AF Simoes
University of Queensland, Brisbane, Australia
You can also search for this author in PubMed Google Scholar
Corresponding author
Correspondence to Taneli Puumalainen .
Additional information
Competing interests.
SL, THK, BQ, EAL, PR, ML have no conflict of interest. TP has worked since October 2007 as a part-time medical advisor for GlaxoSmithKline. HN has received honoraria from GlaxoSmithKline Biologicals for consultancies in the past 3 years. ES has received research grants from Pneumo ADIP, WHO, sanofi pasteur and Wyeth Inc, and has received honoraria from Wyeth Inc and GlaxoSmithKline Biologicals. IR has no conflicts of interest.
Authors' contributions
TP, BQ, THK and EAL conducted the retrospective review of clinical data. TP, SL, BQ and PR designed and monitored collection of clinical data during the vaccine trial. EAL and THK were the clinical study physicians at Bohol Regional Hospital. MGL was the principal investigator of the vaccine trial. HN coordinated the ARIVAC consortium's scientific and administrative activities, and secured the funding. ES and IR were involved in the design of the clinical review process and analysis of it's results. TP, SL, PR, MGL, HN, ES and IR participated in the design of the vaccine trial, development of the analysis plan, and writing of the study report.
All authors have read and approved the final manuscript.
Electronic supplementary material
Additional file 1: table 1. table 2. (doc 80 kb), rights and permissions.
This article is published under license to BioMed Central Ltd. This is an Open Access article distributed under the terms of the Creative Commons Attribution License ( http://creativecommons.org/licenses/by/2.0 ), which permits unrestricted use, distribution, and reproduction in any medium, provided the original work is properly cited.
Reprints and permissions
About this article
Cite this article.
Puumalainen, T., Quiambao, B., Abucejo-Ladesma, E. et al. Clinical case review: A method to improve identification of true clinical and radiographic pneumonia in children meeting the World Health Organization definition for pneumonia. BMC Infect Dis 8 , 95 (2008). https://doi.org/10.1186/1471-2334-8-95
Download citation
Received : 22 November 2007
Accepted : 21 July 2008
Published : 21 July 2008
DOI : https://doi.org/10.1186/1471-2334-8-95
Share this article
Anyone you share the following link with will be able to read this content:
Sorry, a shareable link is not currently available for this article.
Provided by the Springer Nature SharedIt content-sharing initiative
- Lower Respiratory Infection
- Severe Pneumonia
- Acute Lower Respiratory Infection
- Disease Episode
BMC Infectious Diseases
ISSN: 1471-2334
- Submission enquiries: [email protected]
- General enquiries: [email protected]
Thank you for visiting nature.com. You are using a browser version with limited support for CSS. To obtain the best experience, we recommend you use a more up to date browser (or turn off compatibility mode in Internet Explorer). In the meantime, to ensure continued support, we are displaying the site without styles and JavaScript.
- View all journals
- Explore content
- About the journal
- Publish with us
- Sign up for alerts
- Published: 08 April 2021
- Antoni Torres ORCID: orcid.org/0000-0002-8643-2167 1 , 2 , 3 , 4 ,
- Catia Cilloniz ORCID: orcid.org/0000-0002-4646-9838 1 , 2 , 3 , 4 ,
- Michael S. Niederman ORCID: orcid.org/0000-0003-0293-386X 5 ,
- Rosario Menéndez 6 ,
- James D. Chalmers 7 ,
- Richard G. Wunderink ORCID: orcid.org/0000-0002-8527-4195 8 &
- Tom van der Poll 9
Nature Reviews Disease Primers volume 7 , Article number: 25 ( 2021 ) Cite this article
206k Accesses
225 Citations
695 Altmetric
Metrics details
- Respiratory tract diseases
Pneumonia is a common acute respiratory infection that affects the alveoli and distal airways; it is a major health problem and associated with high morbidity and short-term and long-term mortality in all age groups worldwide. Pneumonia is broadly divided into community-acquired pneumonia or hospital-acquired pneumonia. A large variety of microorganisms can cause pneumonia, including bacteria, respiratory viruses and fungi, and there are great geographical variations in their prevalence. Pneumonia occurs more commonly in susceptible individuals, including children of <5 years of age and older adults with prior chronic conditions. Development of the disease largely depends on the host immune response, with pathogen characteristics having a less prominent role. Individuals with pneumonia often present with respiratory and systemic symptoms, and diagnosis is based on both clinical presentation and radiological findings. It is crucial to identify the causative pathogens, as delayed and inadequate antimicrobial therapy can lead to poor outcomes. New antibiotic and non-antibiotic therapies, in addition to rapid and accurate diagnostic tests that can detect pathogens and antibiotic resistance will improve the management of pneumonia.
Similar content being viewed by others
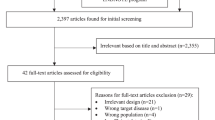
Clinical features for diagnosis of pneumonia among adults in primary care setting: A systematic and meta-review
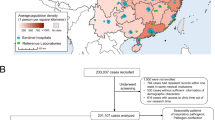
Etiological and epidemiological features of acute respiratory infections in China
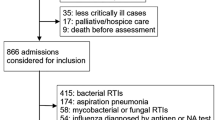
Respiratory viral infections in pragmatically selected adults in intensive care units
Introduction.
Pneumonia is a common acute respiratory infection that affects the alveoli and distal bronchial tree of the lungs. The disease is broadly divided into community-acquired pneumonia (CAP) or hospital-acquired pneumonia (HAP, which includes ventilation-associated pneumonia (VAP)) (Box 1 ). Aspiration pneumonia represents 5–15% of all cases of CAP; however, its prevalence amongst patients with HAP is not known 1 . The lack of robust diagnostic criteria for aspiration pneumonia may explain why the true burden of this type of pneumonia remains unknown 1 .
The causative microorganisms for CAP and HAP differ substantially. The most common causal microorganisms in CAP are Streptococcus pneumoniae , respiratory viruses, Haemophilus influenzae and other bacteria such as Mycoplasma pneumoniae and Legionella pneumophila . Conversely, the most frequent microorganisms in HAP are Staphylococcus aureus (including both methicillin-susceptible S. aureus (MSSA) and methicillin-resistant S. aureus (MRSA)), Enterobacterales, non-fermenting gram-negative bacilli (for example, Pseudomonas aeruginosa ), and Acinetobacter spp. 2 , 3 . In health-care-associated pneumonia (HCAP), owing to patient risk factors, the microbial aetiology is more similar to that in HAP than to that in CAP. However, difficulties in standardizing risk factors for this population, coupled with the heterogeneity of post-hospital health care worldwide, suggest that the concept of HCAP has little usefulness, and indeed, HCAP was not included in recent guidelines for CAP and HAP 3 , 4 , 5 .
Differences in microbiology between CAP and HAP depend on whether pneumonia was acquired in the community or health care environment and on host risk factors, including abnormal gastric and oropharyngeal colonization. In addition, the aetiopathogenesis of CAP is different from that of HAP. In general, mild CAP is treated on an outpatient basis, moderately severe CAP in hospital wards, and severe CAP in intensive care units (ICUs) with or without mechanical ventilation 6 . The need for mechanical ventilation is used as a sub-classification of interest for prognosis and stratification in randomized clinical trials.
Both CAP 7 and HAP 4 can occur in either immunosuppressed or immunocompetent patients. To date, most research data have been based on studies of immunocompetent patients and, therefore, we rely on such sources in this Primer. However, CAP, HAP and VAP in immunosuppressed patients have attracted the attention of researchers, and more investigation is to come.
In this Primer, we cover and summarize the most important and recent updates related to epidemiology, pathophysiology, diagnostic screening, prevention, management, quality of life, and research perspectives. Additionally, owing to the profound impact of the coronavirus disease 2019 (COVID-19) pandemic caused by severe acute respiratory syndrome (SARS) coronavirus 2 (SARS-CoV-2), we summarize the main features of SARS-CoV-2 pneumonia (Box 2 ).
Box 1 Classifications of pneumonia
Community-acquired pneumonia (CAP)
Pneumonia acquired outside the hospital in individuals who have not been hospitalized during the month prior to symptom onset.
Hospital-acquired pneumonia (HAP)
Pneumonia acquired after at least 2 days of hospitalization and when no suspicion of disease incubation before hospital admission is present.
Ventilator-associated pneumonia (VAP)
HAP occurring >48 h after endotracheal intubation.
Aspiration pneumonia
Pneumonia occurring as a result of inhalation of contents from the stomach or mouth into the lungs. It is best considered as part of the continuum between CAP and HAP, and not as a distinct entity.
Health-care-associated pneumonia (HCAP)
Pneumonia acquired in non-hospital care institutions.
Box 2 COVID-19 features
Frequent symptoms
Shortness of breath
Less-common symptoms
Hyposmia (decreased sense of smell) and hypogeusia (decreased sense of taste)
Sore throat
Rhinorrhoea (runny nose)
Muscle pain
Diarrhoea and vomiting
Main complications
Acute respiratory distress syndrome (ARDS)
Sepsis and septic shock
Multiple organ failure
Secondary infection
Epidemiology
Global incidence.
Data from the 2019 Global Burden of Diseases (GBD) study 8 showed that lower respiratory tract infections (LRTIs) including pneumonia and bronchiolitis affected 489 million people globally. Children of <5 years of age and adults of >70 years of age are the populations most affected by pneumonia, according to the 2019 GBD study 8 . In 2019, there were 489 million incident cases of LRTI, and 11 million prevalent cases of LRTI. In the 2016 GBD study, the global incidence of LRTI was 155.4 episodes per 1,000 adults of >70 years of age and 107.7 episodes per 1,000 children of <5 years of age 9 . Finally, aspiration pneumonia contributes 5–15% of all cases of CAP and is associated with worse outcomes, especially in older patients with multiple comorbidities 10 , 11 . There is a lack of data about the incidence of aspiration pneumonia in patients with HAP 1 , 12 .
In the USA, the Etiology of Pneumonia in the Community (EPIC) study 13 found that the annual incidence of CAP was 2.4 cases per 1,000 adults, with the highest rates amongst adults of 65–79 years of age (6.3 cases per 1,000 individuals) and those of ≥80 years of age (16.4 cases per 1,000 people). In Europe, the annual incidence of CAP has been estimated at 1.07–1.2 cases per 1,000 people, increasing to 14 cases per 1,000 people amongst those of ≥65 years of age and with a preponderance in men 14 . Differences in epidemiology between the USA and Europe might be explained by the higher proportion of the adult population who received the pneumococcal vaccine in the USA (63.6% of adults of ≥65 years of age, compared with pneumococcal vaccination rates of 20% to 30% in most European countries 15 , 16 ); in addition, in 2015 in the USA, ~69% of adults of ≥65 years of age had received an influenza vaccine within the previous 12 months. Another possible contributing factor is the decreased rate of smoking in the USA: between 2005 and 2016, the percentage of smokers who quit increased from 51% to 59% 17 . Finally, marked differences between US and European health systems can influence epidemiological data.
The South American Andes region had the highest incidence of adults of >70 years of age with LRTIs (406.5 episodes per 1,000 people), while South Asia had the greatest number of LRTI episodes amongst adults of >70 years of age. Incidence per global region was 171.1 per 1,000 people in Central Europe, eastern Europe and central Asia; 234.4 per 1,000 people in Latin America and the Caribbean; 130.8 per 1,000 people in Southeast Asia, eastern Asia and Oceania; 246.6 per 1,000 people in North Africa and the Middle East; and 229.3 per 1,000 people in sub-Saharan Africa 9 .
According to the 2016 GBD study 9 , Oceania had the highest incidence of LRTI in children (171.5 per 1,000 children of <15 years of age), while South Asia had the greatest number of LRTI episodes amongst children of <5 years of age. Incidence per global region was: 107.1 per 1,000 children in Central Europe, eastern Europe, and central Asia; 94.9 per 1,000 children in Latin America and the Caribbean; 120.4 per 1,000 children in Southeast Asia, eastern Asia and Oceania; 133.2 per 1,000 children in North Africa and the Middle East; and 100.6 per 1,000 children in sub-Saharan Africa.
The epidemiology of pneumonia is constantly changing, owing to the development of molecular diagnostic tests, novel antimicrobial therapies and implementation of preventive measures. Since the beginning of the 21st century, pneumonia has been the most common cause of pandemic infections that have effects on its own epidemiology. In the 2009 influenza pandemic, the influenza virus A H1N1 infected ~200 million people and caused almost 250,000 deaths, with infectivity higher in children than in adults 18 . By contrast, in the current SARS-CoV-2 pandemic, 106 million people had been infected and >2 million had died worldwide by 9 February 2021. However, unlike the influenza virus A H1N1, SARS-CoV-2 affects adults more often than children 19 .
The annual incidence of HAP in adults ranges from 5 to 10 cases per 1,000 hospital admissions globally, whereas VAP affects 10–25% of all patients on mechanical ventilation 3 . HAP is the second most frequent hospital infection after urinary tract infection, and VAP is the most common cause of nosocomial infection and death in the ICU 3 , 4 . The incidence of HAP is highest amongst immunocompromised, post-surgical and older patients 20 . In the USA, the incidence of VAP is estimated to range from 2 to 6 cases per 1,000 ventilator-days 21 , and the incidence of non-ventilator-associated HAP is estimated to be 3.63 cases per 1,000 patient-days 22 . A 2018 systematic review and meta-analysis of studies of VAP in adults from 22 Asian countries found an overall incidence of 15.1 cases per 1,000 ventilator-days 23 . In 2015, data from the prospective French multicentre OUTCOMEREA database (1996–2012) indicated that the risk of VAP was ~1.5% per ventilator-day, decreasing to <0.5% per day after 14 days of mechanical ventilation 24 .
The 2019 GBD study 8 showed that LRTI was responsible for >2.49 million deaths, with mortality highest amongst patients of >70 years of age (1.23 million deaths). These data indicate that mortality due to LRTI is higher than mortality due to tuberculosis (1.18 million deaths) and HIV (864,000 deaths), making it the leading cause of infectious disease mortality worldwide. Indeed, data from a systematic review and meta-analysis on the global and regional burden of hospital admissions for pneumonia estimated that 1.1 million pneumonia-related hospital deaths occurred in 2015 amongst older adults 25 .
In 2016, the highest LRTI mortality rates amongst children of <5 years of age were in the Central African Republic (460 deaths per 100,000 children), Chad (425 deaths per 100,000) and Somalia (417 deaths per 100,000) 9 . Interestingly, data from the 2017 GBD study 26 showed that mortality due to LRTI decreased by 36.4% between 2007 and 2017 for children of <5 years of age, whereas it increased by an estimated 33.6% in adults of ≥70 years of age. LRTI-related deaths amongst children have substantially reduced as a result of the implementation of vaccines (against S. pneumoniae and H. influenzae ), antibiotic therapy, the continuous improvements in education, nutrition, water, sanitation and hygiene, and female empowerment. Nevertheless, in many areas the progress is slow; Nigeria, India, Pakistan, Ethiopia and the Democratic Republic of Congo are the five countries with the highest child mortality 27 .
Conversely, the increased mortality in adults of >70 years of age might be associated with the increasing longevity of the frail older population, chronic diseases, comorbidities 28 , multiple medication use and functional disability, especially in high-income countries. In low-income countries, the high mortality is associated with the effect of air pollution; smoke and alcohol consumption are the main risk factors for pneumonia in this age group.
Globally, amongst children and adults, mortality in those with CAP is related to the treatment setting: <1% in outpatient care, ~4–18% in hospital wards and up to 50% in the ICU 29 , 30 , 31 . However, in adults, age and comorbidities influence mortality. A study that investigated the effects of age and comorbidities on CAP mortality found a mortality of 5% in patients of <65 years of age, 8% amongst patients of 65–79 years and 14% amongst patients of ≥80 years of age 32 , and these rates increased to 20%, 42% and 43%, respectively, in patients with more than one comorbidity. On the basis of studies on long-term mortality across 1–10 years 33 , 34 , 35 , approximately one in three adults will die within one year of being hospitalized with CAP 36 . The estimated in-hospital mortality in patients with chronic obstructive pulmonary disorder (COPD) and CAP has been reported to be 6% during hospitalization and 12%, 24% and 33% within 30 days, 6 months and 1 year from discharge, respectively 37 . Interestingly, 30-day mortality amongst those with pneumococcal pneumonia remained fairly stable in a 20-year study 33 , and this was further confirmed in a review on the burden of pneumococcal CAP in Europe 38 .
Globally, HAP and VAP are considered the leading causes of death due to hospital-acquired infection 39 , 40 , 41 . The estimated global mortality due to HAP is 20–30%, whereas global mortality due to VAP is 20–50% 20 , 42 . Mortality due to VAP in the USA was ~13% 4 . By contrast, a prospective study in central Europe 43 indicated that 30-day mortality due to VAP was 30%. In a large French cohort of patients admitted to the ICU for >48 h, both non-ventilator-associated HAP and VAP were associated with an 82% and a 38% increase in the risk of 30-day mortality, respectively 44 . However, analysis of data from trials on antibiotic therapy for bacterial HAP and VAP to characterize all-cause mortality showed that mortality differed notably within and across studies; all-cause mortality at day 28 was 27.8% in bacterial HAP, 18% in bacterial VAP and 14.5% in non-ventilation-associated bacterial HAP 45 .
In a systematic review and meta-analysis 10 , aspiration pneumonia was significantly associated with increased in-hospital mortality (relative risk 3.62) and 30-day mortality (relative risk 3.57) in patients with CAP treated outside of the ICU. One of the largest studies in aspiration pneumonia demonstrated that mortality in patients with aspiration pneumonia (29%) was more than twice that in patients with CAP (12%) 11 .
Risk factors and differences in epidemiology
Children of <5 years of age 46 and older adults 13 , particularly those of of ≥65 years of age and with comorbidities 14 , 47 , have an increased risk of CAP (Table 1 ). In children, prematurity, malnutrition, household air pollution, ambient particulate matter or suboptimal breastfeeding are the main CAP-related risk factors 48 . In adults, respiratory disease (for example, COPD), diabetes mellitus, cardiovascular disease and chronic liver disease are the most frequent comorbidities that increase the risk of CAP 14 . Of note, men have a higher risk of CAP than women, which may be explained by differences in anatomy, and behavioural, socioeconomic and lifestyle factors 49 .
A US study on the incidence, outcomes and disease burden in >18,000 hospitalized patients with COPD 37 found that, during the 2-year study, 3,419 patients had pneumonia; the annual incidence for CAP was 93.6 cases per 1,000 in the COPD population. In patients without COPD, the incidence was 5.09 cases per 1,000. In the USA, 506,953 adults with COPD are estimated to be hospitalized every year due to pneumonia 37 .
Immunocompromised patients have a higher risk of CAP than the general population 7 , 14 . A secondary analysis of an international, multicentre study from 54 countries worldwide found that almost one in five patients hospitalized with CAP were not immunocompetent 7 . Amongst patients with CAP, 18% had one or more risk factors for immunodeficiency, with chronic steroid use (45%), haematological cancer (25%) and chemotherapy (22%) being the most frequent.
Several studies have also demonstrated an association between lifestyle factors and the risk of CAP, including smoking, high alcohol consumption, being underweight (owing to under-nutrition or underlying conditions that compromise the immune response), living conditions, such as a large household or regular contact with children, and others 14 . Smoking is associated with colonization by pathogenic bacteria and an increased risk of lung infection, especially by S. pneumoniae 50 . Consumption of 24 g, 60 g and 120 g of pure alcohol daily (one standard alcoholic beverage equals 10 ml or 8 g of pure alcohol, and it is the approximate amount of alcohol that the average adult can process in an hour) resulted in relative risks for CAP of 1.12, 1.33 and 1.76, respectively, compared with no consumption 51 . In addition, exposure to air pollution may increase the risk of pneumonia in the short and long term; a study in 345 hospitalized patients with CAP and 494 controls (patients who were admitted in the same period but for non-pneumonia reasons) demonstrated that long-term exposure (1–2 years) to high levels of air pollutants (particulate matter 2.5 μm and nitrogen dioxide) was associated with increased hospitalization in those of ≥65 years of age 52 .
Factors that increase the risk of HAP can be categorized into patient-related and treatment-related groups (Table 1 ). Oropharyngeal colonization is the main mechanism underlying HAP. However, much attention has been shifted to oropharyngeal colonization in critically ill patients (present at ICU admission or occurring during ICU stay) 53 . A study from Japan investigating oral colonization in residents in long-term care facilities found that 38% of these individuals were colonized with antibiotic-resistant pathogens, mainly Acinetobacter spp., Enterobacterales and Pseudomonas spp. The presence of these pathogens represents a potential risk for pneumonia 54 . Indeed, current international guidelines have suggested that previous colonization by antibiotic-resistant pathogens be considered when identifying patients with an increased risk of HAP due to such pathogens 3 , 4 .
Colonization and biofilm formation were present within 12 h of intubation and remained for >96 h in most patients 55 . Underpinning an important association between intubation and VAP pathogenesis, this study also showed that colonization in patients undergoing mechanical ventilation occurred in the oropharynx and stomach first, followed by the lower respiratory tract and, thereafter, the endotracheal tube 55 . Intubation and mechanical ventilation can increase the risk of developing VAP by 6–21-fold, with the highest risk within the first 5 days of intubation 53 . Endotracheal tubes enable the direct entry of bacteria into the lower respiratory tract, interfere with normal host defence mechanisms and serve as a reservoir for pathogenic microorganisms.
Multiple risk factors are related to aspiration pneumonia, each one increasing the chance of gastric contents reaching the lungs. The most frequent of these factors are impaired swallowing, decreased consciousness and an impaired cough reflex 1 (Table 1 ).
Microbial aetiology
Knowledge of pathogens associated with pneumonia is crucial to provide more targeted empiric antibiotic therapy, prevent the emergence of antimicrobial resistance through selection pressure and reduce health-care-associated costs.
The microbial aetiology of CAP differs by its severity at clinical presentation and by season 2 , 56 , 57 , 58 . However, the microbial aetiology of CAP is not detected in ~50% of patients; possible reasons include the failure to obtain a respiratory sample adequate for culture or before the initiation of antibiotic therapy and the inconsistent availability of newly improved molecular tests 59 . S. pneumoniae remains the most frequent pathogen in CAP, although a study in North America found that its incidence has decreased owing to the introduction of polysaccharide vaccines 60 and a reduced smoking rate 61 , 62 . No such decrease has been observed in Europe 2 , 63 , 64 , 65 (Fig. 1 ).
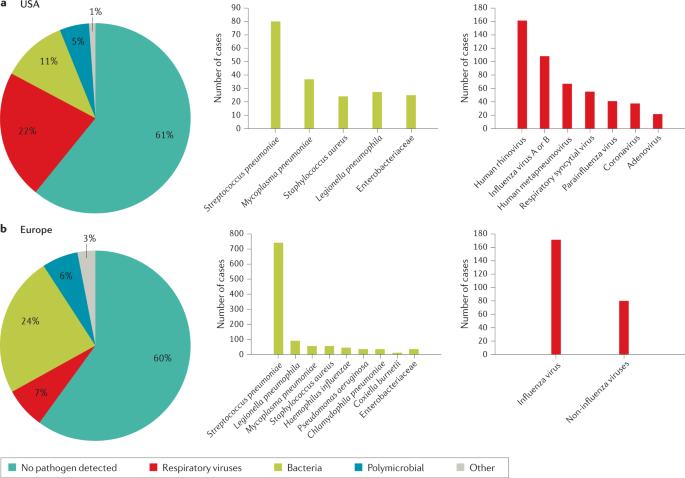
a | Aetiology of community-acquired pneumonia (CAP) in the adult population in the USA from 2010 to 2012 (from 2,488 cases) 9 . b | Aetiology of CAP in the adult population in Europe from 2003 to 2014 (from 3,854 cases) 6 . Possible reasons that may explain the challenge in identifying the aetiology of pneumonia include difficulty in obtaining samples from the lower respiratory tract, the effect of antibiotic use prior to sample collection and low sensitivity of some diagnostic tests.
In a small proportion of patients, CAP is caused by MRSA and antibiotic-resistant gram-negative bacteria (for example, P. aeruginosa and Klebsiella pneumoniae ) 2 , 66 . As antibiotic resistance complicates clinical management, clinicians need to recognize risk factors for these pathogens and initiate adequate empirical therapy in response (Box 3 ). The main risk factors for multidrug-resistant (MDR) pathogens in CAP include immunosuppression, previous antibiotic use, prior hospitalization, use of gastric acid-suppressing agents, tube feeding and non-ambulatory status 67 . Various scoring systems can help to determine the risk of infection by antibiotic-resistant pathogens.
The P. aeruginosa , extended-spectrum β-lactamase (ESBL)-positive Enterobacterales and MRSA (PES) score 68 is based on several risk factors, including age 40–65 years and male sex (one point each), age >65 years, previous antibiotic use, chronic respiratory disorder and impaired consciousness (two points each), and chronic renal failure (three points). The PES score has been validated in general wards, ICUs and a very old population (age ≥80 years). One study 69 demostrated that there is an 80% probability of detecting a PES pathogen with the PES score, demonstrating good accuracy of the score. In another study 70 , the accuracy of the PES score in patients of ≥80 years of age with CAP was ~64%, highlighting differences in clinical characteristics of this population who are more susceptible to infections, recurrent pneumonia and sepsis.
The drug resistance in pneumonia (DRIP) score 71 is based on both major and minor risk factors. Major risk factors (two points each) include previous antibiotic use, residence in a long-term care facility, tube feeding and prior infection by a drug-resistant pathogen (within the past year). Minor risk factors (one point each) include hospitalization within the previous 60 days, chronic pulmonary disease, poor functional status, gastric acid suppression, wound care and MRSA colonization (within the past year).
The use of new diagnostic molecular techniques has led to an increased interest in the role of respiratory viruses as potential aetiological agents in CAP. Recent studies have reported that respiratory viruses account for 7–36% of CAP cases with a defined microbial aetiology 13 , 72 , 73 . A recent study from China reported that in patients with viral CAP, influenza virus, non-influenza virus and mixed viral infections were the cause of CAP in 63%, 27% and 10% of patients, respectively (Fig. 2 ). The outcomes were similar between patients with CAP due to influenza virus and those with CAP due to non-influenza viruses, although in patients with CAP due to non-influenza viruses the incidence of complications was higher 74 . In another study, 3% of all patients with a diagnosis of CAP admitted to the emergency department had pure viral sepsis 75 . Viral sepsis was present in 19% of those admitted to ICU, and sepsis was present in 61% of all patients with viral CAP.
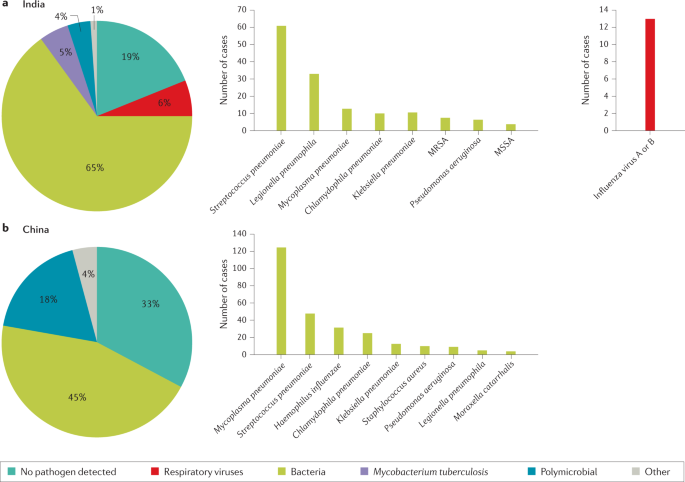
a | Aetiology of community-acquired pneumonia (CAP) in the adult population in India from 2013 to 2015 (from 225 cases) 54 . b | Aetiology of CAP in the adult population in China from 2004 to 2005 (from 593 cases) 55 . Possible reasons that may explain the challenge in identifying the aetiology of pneumonia include difficulty in obtaining samples from the lower respiratory tract, the effect of antibiotic use prior to sample collection and low sensitivity of some diagnostic tests. MRSA, methicillin-resistant Staphylococcus aureus ; MSSA, methicillin-susceptible Staphylococcus aureus .
Respiratory viruses are detected in more than half of children with CAP 76 . Respiratory viruses were the most frequent cause of pneumonia (66%) in children with an aetiological diagnosis in the USA, with respiratory syncytial virus, rhinovirus and metapneumovirus being the most common ones 76 . Bacterial pathogens were the cause of CAP in 8% of patients, with S. pneumoniae and S. aureus being the most common bacteria. Bacteria–virus co-infections were detected in 7% of patients.
Box 3 Pathogen-specific risk factors
Streptococcus pneumoniae : Dementia, seizure disorders, congestive heart failure, cerebrovascular disease, chronic obstructive pulmonary disease (COPD), HIV infection, overcrowded living conditions and smoking
Legionella pneumophila : Smoking, COPD, compromised immune system, travel to outbreak areas, residence in a health-care facility and proximity to cooling towers or whirlpool spas
MRSA : Previous MRSA infection or colonization, residence in a nursing home or long-term care facility and prior hospitalization within the previous 90 days
Pseudomonas aeruginosa : Pulmonary comorbidity
Enterobacterales: Residence in a nursing home
MRSA, methicillin-resistant Staphylococcus aureus
Data on microbial aetiology of HAP have mostly been obtained from patients with VAP. However, studies in patients with HAP or VAP with known microbial aetiology have shown that both HAP and VAP have similar microbial aetiology, with P. aeruginosa and S. aureus being the most frequent pathogens. Other pathogens such as Acinetobacter spp. and Stenotrophomonas spp. are more frequently reported in VAP 4 , 77 .
Antibiotic resistance is the main concern with HAP and VAP. Assessing risk factors for MDR organisms (resistant to at least one agent in three or more groups of antibiotics), extensively drug-resistant organisms (XDR; resistant to one or more agents in all but one or two antibiotic groups) and pandrug-resistant organisms (resistant to almost all groups of approved antibiotics) is central to managing patients with these pathogens 78 . In general, we can classify the risk into three categories: (1) local epidemiology (for example, ICU with high rates of MDR pathogens); (2) patient risk factors (including structural pulmonary diseases (for example, bronchiectasis), antibiotic use during the 90 days prior to HAP or VAP onset, hospitalization (2–5 days) during the 90 days prior to HAP or VAP onset, septic shock at VAP onset, acute respiratory distress syndrome (ARDS) preceding VAP, at least 5 days of hospitalization prior to VAP onset, and acute renal replacement therapy prior to VAP onset) 42 ; and (3) previous colonization or infection with MDR pathogens 42 . Anaerobes and gram-negative bacilli (for example, E. coli , K. pneumoniae and P. aeruginosa ) are the most frequent microorganisms found in aspiration pneumonia 1 .
Mechanisms/pathophysiology
From colonization to infection.
The mechanisms that drive LRTIs have become increasingly known. Most instances of bacterial pneumonia are caused by microorganisms that translocate from the nasopharynx to the lower respiratory tract 79 , 80 . Bacteria enter the nasopharynx after shedding from a colonized individual. Pathogens can spread between individuals via direct or indirect contact, droplets and aerosols 81 . Transmission success depends on many variables, including environmental conditions, gathering of people and host factors, such as the distribution of pattern recognition receptors in the epithelial cells of the airways 81 . Pathogen adherence to the upper airway epithelium is a crucial first step in colonization and subsequent infection. Once in the nasopharynx, bacteria escape from mucus and attach to the epithelium using multiple strategies to evade host clearance, including expression of host-mimicking or antigenically varying molecules 82 (that is, molecules that imitate the structure of host molecules or can vary their antigens to avoid recognition by host immune cells). Microorganisms gain entry to the lower airways through inhalation or, less frequently, by pleural seeding from blood. Selection of colonizing mutants that can evade immune clearance is considered to precede infection 79 . Infection occurs when host defences are impaired and/or there has been exposure to a highly virulent microorganism or a large inoculum. Several factors can facilitate the transition from colonization to infection, including preceding viral infection and chronic lung diseases. Other mechanisms involved in the increased susceptibility to infection include loss of barrier integrity and impaired host defences due to complex interactions amongst anatomical structures, microorganisms (and their virulence factors) and the host immune system 79 , 80 , 83 .
Of note, it has become clear that healthy lungs are not sterile; instead, they harbour a unique microbiota that includes ~100 different taxa 84 . The main genera in healthy lower airways are Prevotella , Streptococcus , Veillonella , Fusobacterium and Haemophilus 84 . The pathogenesis of pneumonia has been suggested to include a change in the lung microbiota, from a physiological, homeostatic state to dysbiosis, in association with a low microbial diversity and high microbial burden, and with corresponding immune responses 84 , 85 To further support this concept, longitudinal lung microbiota studies are required to document transitions from homeostatic to dysbiotic states during the development and resolution of pneumonia. An additional area of research lies in analysing the virome and mycobiome in airways and their influence on host defence against pneumonia. The mechanisms by which lung microbiota affect immunity in the airways have been partially elucidated. Bacteria present in the upper airways that potently stimulate nucleotide-binding oligomerization domain-containing (NOD)-like receptors ( Staphylococcus aureus and Staphylococcus epidermidis ) increase resistance to pneumonia through NOD2 and induction of release of granulocyte–macrophage colony-stimulating factor 86 .
Mechanisms of infection
A general mechanism of infection of the lower airways is difficult to define. The many different microorganisms that can cause pneumonia do not seem to express specific features. Even in specific populations (for example, young children, hospitalized patients, older individuals), a spectrum of pathogens, rather than a specific microorganism, can cause pneumonia. This finding has led to the assumptions that the development of pneumonia largely depends on the host response to the microbe in the airways, with pathogen characteristics playing a less prominent role 83 . Nonetheless, virulence factors expressed by microorganisms do contribute to the ability of specific pathogens to cause pneumonia 79 , 80 . For example, pneumolysin, a virulence factor expressed by S. pneumoniae , is a member of the cholesterol-dependent cytolysin family that can form large pores in (and thereby injure) eukaryotic cells with cholesterol-containing membranes 87 . S. aureus expresses several virulence factors, such as α-haemolysin (also known as α-toxin), a pore-forming toxin that causes cell death via activation of the inflammasome 88 . α-Haemolysin binds to the disintegrin and metalloproteinase domain-containing protein 10 (ADAM10) and results in disruption of the barrier function of the respiratory epithelium 88 . Finally, toxins secreted by the type III secretion system are a key element in P. aeruginosa virulence in the lung. Genes encoding type III-secreted toxins are induced in P. aeruginosa upon contact with host cells, eliciting a plethora of effects, including cytotoxicity 89 .
Once an LRTI has occurred, the maintenance of lung homeostasis whilst in the presence of microbes depends on an adequate balance between two seemingly opposing processes, immune resistance and tissue resilience, that are largely mediated by the same cell types. Whilst immune resistance seeks to eliminate invading microbes, tissue resilience strives to prevent or resolve tissue damage caused by the immune response, the pathogen or both 83 . The organized actions of immune resistance and tissue resilience determine whether and how an LRTI progresses or resolves. Inadequate or unfitting immune responses can result in adverse outcomes, such as ARDS, defined as the acute onset of non-cardiogenic pulmonary oedema, hypoxaemia and the need for mechanical ventilation 90 , 91 . Unbalanced immune responses during pneumonia can also result in extrapulmonary complications, some of which can occur up to years after the respiratory illness (see below).
Immune resistance
Anatomical barriers present the first line of defence against pneumonia. Mucociliary clearance, mediated by mucous and liquid layers and cilia on the surface of respiratory epithelial cells, is considered the primary innate defence mechanism 92 . The respiratory epithelium produces a robust barrier composed of secretory products, surface glycocalyces and membranes, and intercellular junctional proteins linked to the actin cytoskeleton 92 . Cell-associated and secreted mucins form a polymeric glycoconjugate layer that can bind and transport pathogens from the airways 92 . The branching bronchial tree provides an additional defence mechanism by preventing particles of >3 µm in diameter from entering the lower airways 92 . If microbes do reach the lower respiratory tract, the host defence becomes shaped by an interplay between resident and recruited immune cells and mechanisms (Fig. 3 ).
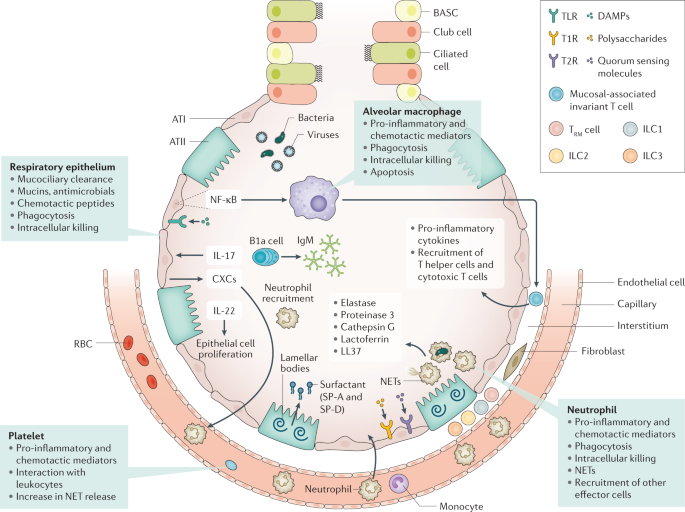
Immune resistance aims to eradicate microorganisms that invade the airways. Respiratory epithelial cells are covered by cell-associated and secreted mucins that form a layer of polymeric glycoconjugates that remove pathogens from the airways. The epithelium can also remove pathogens through phagocytosis and intracellular killing. The quiescent alveolar space contains many alveolar macrophages that, upon activation, can phagocytose and kill pathogens, which is improved by apoptosis. Innate lymphoid cells (ILCs) are tissue-resident cells populating the pulmonary mucosa. Together with natural killer cells, ILCs boost host defence during airway infection. Neutrophils migrate to the airways attracted by chemotactic proteins released by respiratory epithelial cells and alveolar macrophages; these chemotactic proteins also promote the recruitment of other leukocyte subsets. The lung contains a marginated pool of neutrophils tethered to the vasculature, enabling rapid neutrophil recruitment into tissue upon infection. Adequate pulmonary immunity entails neutrophil-mediated killing of invading microbes by several effector mechanisms, including the release of neutrophil extracellular traps (NETs). Platelets can form complexes with leukocytes, facilitating NET formation and the release of microbicidal agents. Resident memory T (T RM ) cells are generated after exposure to pathogens and reside in the quiescent lung. ATI, alveolar type I cell; ATII, alveolar type II cell; BASC, bronchioalveolar stem cell; CXCs, CXC chemokines; DAMPs, damage-associated molecular patterns; NF-κB, nuclear factor-κB; RBC, red blood cell; SP, surfactant protein; T1R, G-protein-coupled sweet taste receptor; T2R, G-protein-coupled bitter taste receptor; TLR, Toll-like receptor.
Innate immunity
Various innate immune cells reside in quiescent airways to provide the next line of defence against pathogens. Lung epithelial cells can be triggered through a variety of receptors that recognize not only pathogens but host-derived molecules as well, including damage-associated molecular patterns (released upon cell injury) and cytokines. Many pattern recognition receptors (for example, toll-like receptors) then induce nuclear factor ĸB, which is a major driver of protective immunity in the epithelium 93 , 94 . In the alveoli, surfactant proteins SP-A and SP-D produced by type II epithelial cells can directly inhibit microbes 95 . Recently, G-protein-coupled bitter taste receptors (T2R) and sweet taste receptors (T1R) were identified in respiratory epithelial cells 96 ; bacterial quorum-sensing molecules can trigger bitter taste receptors, whilst sugars can activate sweet receptors, and these interactions may then modify host defence mechanisms 97 . IL-17 and IL-22 mediate protection during pneumonia largely through epithelial cell activation 98 . IL-17 stimulates the epithelium to secrete antimicrobial proteins and CXC chemokines that trigger neutrophil recruitment. The protective properties of IL-22 are linked to its function in stimulating epithelial cell proliferation, which is indispensable for repair following injury 99 .
Alveolar macrophages (AMs), which reside on lower airway surfaces, have essential roles in both immune resistance and tissue resilience 100 . During homeostasis, they limit the effect of potentially noxious environmental stimuli through anti-inflammatory effects. The crucial role of AMs in immune resistance during pneumonia is illustrated by studies showing impairment of the host defence when AM function is disrupted 94 . Microbes can activate AMs via several pattern recognition receptors and nuclear factor ĸB, leading to the production of pro-inflammatory cytokines that orchestrate subsequent, innate immune responses necessary for resistance. In addition, stimulated by AM apoptosis, activated AMs can phagocytose and kill pathogens 101 . By contrast, AM death via non-apoptotic pathways, such as necroptosis, impairs antibacterial defence during pneumonia 102 . The complex role of necroptosis in the host response to bacterial infection is illustrated by reports linking necroptosis to exaggerated inflammation and impaired bacterial clearance during S. aureus pneumonia 103 , whereas it has a protective, anti-inflammatory effect associated with improved bacterial clearance during systemic S. aureus infection 104 . Local conditions may instruct AMs in providing the most suitable response.
Innate lymphoid cells (ILCs) serve as counterparts to T cells by regulating immune responses via the production of effector cytokines and by influencing functions of other innate and adaptive immune cells 105 . These cells are especially abundant on the mucosal surfaces of the lung. There are three major groups of ILCs, namely, ILC1, ILC2 and ILC3. ILC classification reflects these cells’ capacity to secrete types 1, 2 and 17 cytokines, respectively. Beneficial roles for ILC1s and ILC2s have been reported in viral pneumonia models 106 , 107 ; lung ILC3s have a protective role in pneumonia by secreting IL-17 and IL-22 (refs 108 , 109 ). Mucosal-associated invariant T cells are other innate-like T lymphocytes that are abundant in the lung mucosa 110 . These cells probably have a role in protective immunity during airway infection through a variety of mechanisms, including production of pro-inflammatory cytokines, macrophage activation and recruitment of effector helper and cytotoxic T cells 111 .
When resident cells are unable to eradicate invading pathogens, mechanisms are activated to attract additional effector cells to the site of infection. Neutrophils are the first and most profusely recruited cells in response to infection 112 . Primed neutrophils have a strongly increased capacity to phagocytose microbes and initiate a respiratory burst response 112 . In addition, neutrophil products, such as elastase, proteinase 3 (also known as myeloblastin), cathepsin G, lactoferrin and LL-37, exert potent antimicrobial activities 113 . Neutrophil extracellular traps, comprising decondensed chromatin fibres that carry histones and antimicrobial peptides, are also released to kill pathogens 113 . The crucial role of neutrophils in pulmonary immune resistance is illustrated by the increased susceptibility found in patients with neutropenia or neutrophil deficiencies and mouse pneumonia models, in which neutrophil depletion has been shown to exacerbate infection with several pathogens 112 . In addition to AMs, newly recruited inflammatory monocytes–macrophages are involved in immune resistance during pneumonia 114 . In mice, induction of K. pneumoniae -associated pneumonia has been found to lead to the recruitment of inflammatory monocytes to the lungs where they mediate the influx of protective ILCs producing IL-17 through the release of tumour necrosis factor 109 . Innate-like B1 B cells mainly reside in the pleural space. In response to infection, B1a B cells migrate to the lung parenchyma to produce polyreactive immunoglobulin M and contribute to protective immunity 115 . Platelets also provide immune resistance during pneumonia through various mechanisms, including platelet–bacteria interactions and complex formation with leukocytes. Other mechanisms include facilitating neutrophil extracellular trap formation and stimulating the release of microbicidal agents that can directly lyse bacteria 116 . Thrombocytopenia is associated with impaired antibacterial defence during murine pneumonia 117 , 118 .
Finally, several distant organs can affect immune resistance in the respiratory tract. For example, depletion of gut microbiota by broad-spectrum antibiotics has been shown to impair host defence during viral and bacterial pneumonia in mice 119 , 120 . This protective gut–lung axis has been hypothesized to be mediated, at least in part, by gut-derived microbial products that can improve host defence mechanisms in other tissue 121 . The existence of a liver–lung axis has been suggested in many studies; pneumonia elicits a robust acute-phase protein response in the liver, probably mediated by cytokines released into circulation, and distinct acute-phase proteins can improve antibacterial defence through several mechanisms, for example, by enhancing opsonophagocytosis (phagocytosis mediated by opsonins) and respiratory burst activity by immune cells and by limiting iron availability to bacteria.
Adaptive immunity
Previous encounters with respiratory pathogens shape memory defence mechanisms against pneumonia. Evidence highlights roles of innate immune cells (for example, epithelial cells and AMs) that had been modified by a prior infection to trigger epigenetic alterations in a so-called process of ‘trained immunity’ 122 . Trained immunity has received attention within the context of pneumonia in humans. The Bacille Calmette–Guérin vaccination induces trained immunity. When administered to older patients after hospital discharge, the vaccination increased time to first infection, and most of the protection was observed against respiratory tract infections of probable viral origin 123 . Humoral response to microbes improves host defence by producing pathogen-specific antibodies, as illustrated by the efficacy of vaccines in diminishing the risk of pneumonia.
The airways contain pools of memory cells that are assembled in tertiary lymphoid organs in the upper airways and in bronchus-associated lymphoid tissue in the lower airways. Together, these cells protect against infection through local and systemic antibody production 124 . The majority of CD4 + T cells and CD8 + T cells in the quiescent lung have a memory phenotype (hence they are named resident memory T (T RM ) cells) and are generated in response to exposure to respiratory pathogens 125 . In experimental mouse models, the lung is enriched with T RM cells specific for multiple viral and bacterial pathogens following a respiratory infection, and these cells contribute to future protective immunity. For example, lobar pneumococcal pneumonia in mice leads to the accumulation of CD4 + T RM cells in the infected lobe, but not in other areas of the lung. This T RM cell-populated lobe expresses better defence against reinfection by S. pneumoniae than other lobes 126 .
Tissue resilience
Tissue resilience is essential in controlling excessive inflammation whilst sustaining effective protection against microbes (Fig. 4 ). AMs contribute to tissue resilience by producing anti-inflammatory cytokines, such as IL-10 and IL-1 receptor antagonist, and through the phagocytosis of apoptotic leukocytes. This process is named efferocytosis and protects tissue in two manners: by preventing the release of pro-inflammatory and toxic contents from dying cells and by concurrently prompting the release of anti-inflammatory and repair factors, including transforming growth factor β1, prostaglandin E 2 , and pro-resolving lipid mediators 100 . Pro-resolving lipid mediators (resolvins, protectins and maresins) can mediate a large variety of immune responses in pneumonia, both increasing bacterial killing and promoting tissue repair 127 . Such mediators have been shown to have important protective roles in mouse models of bacterial pneumonia 128 , 129 .
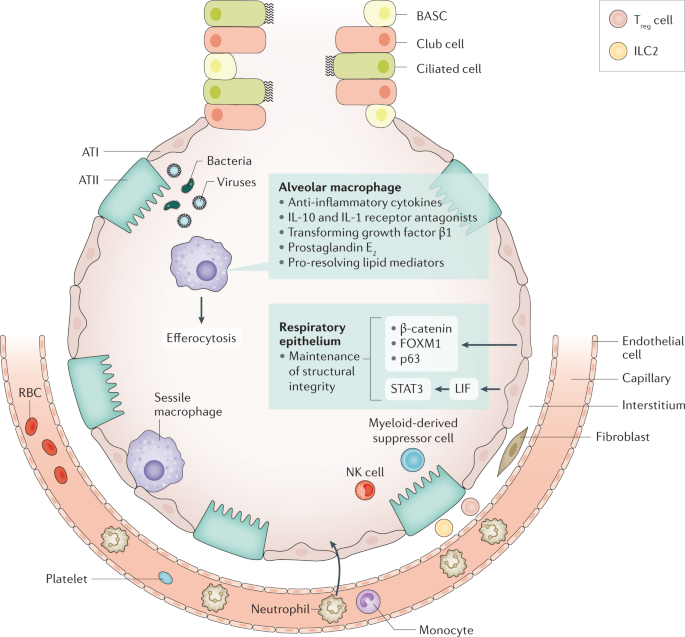
Tissue resilience controls excessive inflammation whilst safeguarding protection against pathogens. The respiratory epithelium is an important player in tissue resilience. Maintenance of the structural integrity of the epithelial barrier is a crucial factor here. Alveolar macrophages also have an important role, via release of anti-inflammatory mediators and efferocytosis (phagocytosis of apoptotic leukocytes). Sessile macrophages adhere to the epithelium, where they probably contribute to tissue resilience. Cell types recruited to the site of infection during pneumonia that are involved in tissue resilience include myeloid-derived suppressor cells, regulatory T (T reg ) cells, type 2 ILC2s and natural killer (NK) cells. ATI, alveolar type I cell; ATII, alveolar type II cell; BASC, bronchioalveolar stem cell; FOXM1, forkhead box protein M1; ILC, innate lymphoid cell; LIF, leukaemia inhibitory factor; RBC, red blood cell; STAT3, signal transducer and activator of transcription 3.
The structural integrity of the epithelial barrier in the respiratory tract is crucial to tissue resilience. Contributors to epithelial resilience include β-catenin (also known as catenin β1) 130 , forkhead box protein M1 (FOXM1) 131 , tumour protein 63 (p63) 132 and signal transducer and activator of transcription 3 (STAT3) 133 , 134 . Interestingly, a deficiency of STAT3 in airway epithelial cells results in exaggerated lung injury during experimental pneumonia 133 , 134 . Epithelial cell-derived leukaemia inhibitory factor (LIF) has been implicated as an important inducer of STAT3 in the respiratory epithelium, and inhibition of LIF has been shown to increase lung injury in pneumonia 135 . Several immune cells recruited to the site of infection during pneumonia are known to contribute to tissue resilience, including myeloid-derived suppressor cells 136 , regulatory T cells 137 , ILC2s 138 and natural killer cells 139 , 140 .
Lung pathology
With respect to the histopathology of bacterial pneumonia, four stages have classically been described: congestion, red hepatization, grey hepatization and resolution (Fig. 5 ). The term hepatization refers to an increased firmness of inflamed lung tissue that renders the tissue consistency similar to that attributed to hepatic tissue. In the early stages of bacterial pneumonia, lung tissue shows mild intra-alveolar oedema and congestion of the capillaries within the alveolar septa 141 . This stage is followed by inflammatory exudation with an accumulation in the alveolar spaces of neutrophils, red blood cells and fibrin, and a subsequent, gradual disintegration of red blood cells and neutrophils. The exudates are eventually transformed into intra-alveolar fibromyxoid moulds, consisting of macrophages and fibroblasts, and gradual resolution follows thereafter.
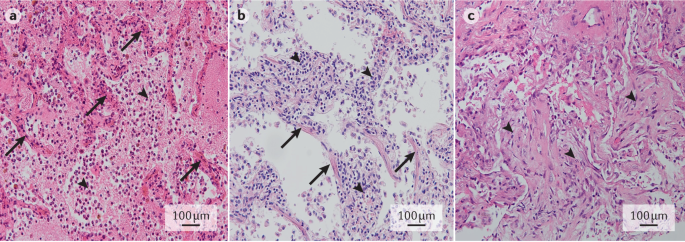
a | Early stage bacterial pneumonia, with congestion of septal capillaries (arrows) and intra-alveolar presence of oedema, neutrophils and a meshwork of fibrin strands (arrowheads). b | Early stage viral pneumonia, with interstitial lymphocytic infiltrates (arrowheads) and diffuse alveolar damage, as evidenced by the presence of hyaline membranes (arrows). c | Organizing pneumonia, with intra-alveolar fibroblast plugs (arrowheads) and few remnant fibrin deposits. Haematoxylin and eosin staining; original magnification ×20. Images in parts a – c courtesy of J.J.T.H. Roelofs, Amsterdam UMC, Netherlands.
Viral pneumonia is typically associated with interstitial inflammation and diffuse alveolar damage 142 . Interstitial inflammation involves the alveolar walls, which widen and usually contain an inflammatory infiltrate of lymphocytes, macrophages and plasma cells in some cases. Alveolar damage is characterized by pink hyaline membranes lining the alveolar septa that follow a pattern of organization and resolution similar to that of intra-alveolar inflammation in bacterial pneumonia.
In addition to these features, specific microorganisms may cause different histopathological changes such as granulomas, multinucleated giant cells or specific viral inclusions.
Extrapulmonary complications
Extrapulmonary complications are extremely common in patients with pneumonia, including those without sepsis. Such complications entail both acute and long-term adverse sequelae. Patients who have been hospitalized for pneumonia have higher rates of all-cause hospitalization and an increased mortality risk for 10 years after discharge 35 compared with matched patients hospitalized for other pneumonia-unrelated conditions.
Sepsis, defined as a life-threating organ dysfunction caused by a dysregulated host response to an infection 143 , is most often caused by pneumonia (up to half of all patients with sepsis) 144 . Conversely, of patients who are hospitalized with CAP 145 or HAP 146 , 36% and 48% have been reported to develop sepsis, respectively. Both pro-inflammatory and anti-inflammatory reactions characterize host response to sepsis, which varies strongly between individuals. Pro-inflammatory responses include the release of cytokines, activation of the complement and coagulation system (which could result in disseminated intravascular coagulation), and disruption of the normal barrier and anticoagulant function of the vascular endothelium. Anti-inflammatory responses can result in immune suppression, in part due to apoptotic loss of lymphoid cells 147 , 148 .
Cardiovascular disease
Pneumonia particularly affects the cardiovascular system, and its effects include depression of left ventricular function, myocarditis, arrhythmias, ischaemia and infarction 149 . Patients hospitalized for pneumonia have an increased short-term and long-term risk (up to ten years) of cardiovascular disease 150 . A meta-analysis of the incidence of cardiac events within 30 days of pneumonia diagnosis found new or worsening heart failure in 14% of all patients, new or worsening arrhythmias in 5% and acute coronary syndromes in 5% 151 . Approximately 90% of cardiac complications occur within 7 days of a pneumonia diagnosis, and more than half occur within the first 24 h 149 . In a multicentre study, one third of patients hospitalized for CAP experienced intrahospital cardiovascular events, mainly involving the heart, and such occurrence was associated with a fivefold increase in 30-day mortality. Independent risk factors for cardiovascular events were severity of pneumonia and pre-existing heart failure 152 . Additionally, hospitalization for pneumonia is associated with an increased risk of new-onset heart failure in the intermediate and long term, with a hazard ratio of 2 after 5 years 34 . In patients with pneumonia who require ICU treatment within 24 h of hospital admission, approximately half have diagnostic criteria for myocardial infarction 153 ; cardiac complications are the direct or main cause of death in 27% of patients hospitalized for pneumonia 154 . Notably, whilst the increased risk for myocardial infarction associated with pneumonia is proportional to disease severity, it is not restricted to patients with pneumonia-induced sepsis 155 . Even mild respiratory infection is associated with an increased risk of myocardial infarction for several months after the onset of infection 155 .
The mechanisms underlying an increased risk of cardiovascular disease after pneumonia are probably multifactorial. Hypoxaemia due to impaired gas exchange and ventilation–perfusion mismatching, as well as endothelial dysfunction causing vasoconstriction, may increase vulnerability to ischaemic events 149 . Systemic inflammation during pneumonia can increase inflammatory activity within coronary atherosclerotic plaques, rendering them prone to rupture 149 . The systemic host response during pneumonia also entails endothelial dysfunction and procoagulant changes, which can promote thrombus formation at the site of a ruptured coronary plaque 149 . Indeed, as reflected by elevated markers of coagulation activation in the circulation, the majority of patients admitted to hospital for pneumonia have a procoagulant phenotype 156 , 157 .
Patients with pneumonia and acute coronary syndromes show higher platelet-aggregating activity than patients with acute coronary syndromes without pneumonia 149 . Notably, the connection between pneumonia and cardiovascular disease is probably bidirectional. For example, pre-existing heart failure is a risk factor for pneumonia, perhaps partially related to impaired immune responses 149 . Preclinical investigations suggest that lung congestion can facilitate the growth of common respiratory pathogens in the airways 149 . With regard to long-term risk, investigations in mice predisposed to developing atherosclerosis 158 and post mortem examinations in humans 159 have suggested that infection can elicit pro-inflammatory responses in atherosclerotic lesions and result in increased vulnerability for coronary and cerebrovascular events. For example, acute lung inflammation induced by intratracheal administration of lipopolysaccharide in mice prone to atherosclerosis resulted in destabilization of atherosclerotic plaques; neutrophil depletion prevented this destabilization, suggesting a role for neutrophils in plaque weakness elicited by lung injury 160 . In addition, systemic inflammation and coagulation are sustained in many patients with pneumonia and have been associated with an increased risk of cardiovascular death 161 , 162 . Left ventricular dysfunction during pneumonia may be secondary to depressant activity of pro-inflammatory cytokines in circulation and/or altered vascular reactivity 149 .
Other complications
Additional extrapulmonary complications of pneumonia include a decline in cognition and functional status 163 , 164 . Pneumonia is associated with a 57% increase in the risk of dementia 164 . Encephalopathy associated with acute infectious disease has been studied in the context of sepsis 165 , 166 . Mechanisms involved include impaired circulation in the brain secondary to hypotension, a disturbed vasoreactivity, endothelial dysfunction and microvascular thrombosis, which can result in ischaemic and haemorrhagic lesions. The blood–brain barrier can be disturbed through increased activity of pro-inflammatory cytokines and reactive oxygen species produced at least in part by astrocytes. Activation of microglia can further contribute to neuronal damage in the brain 166 .
Approximately one fifth of patients hospitalized with pneumonia are readmitted to the hospital within 30 days; pneumonia, cardiovascular disease and (chronic obstructive) pulmonary disease are the most common diagnoses 167 . An increased susceptibility for infection after pneumonia may be related to a relatively immunocompromised state, as has been described in patients with sepsis 147 . Knowledge of immunological defects contributing to recurrent pneumonia (usually defined as a new episode of pneumonia within several months of the previous one, separated by at least a 1-month asymptomatic interval and/or radiographic clearing of the acute infiltrate) 168 is limited. A small study involving 39 patients suggested that immunoglobulin deficiency and an inability to react to polysaccharide antigens are associated with an increased incidence of recurrent pneumonia 169 . Further, a study in mice found a reduced capacity of AMs to phagocytose E. coli and S. aureus following recovery from primary pneumonia, a reduction mediated by signal-regulatory protein-α (also known as tyrosine–protein phosphatase non-receptor type substrate 1) and associated with an impaired host defence after secondary infection of the lower airways 170 .
Diagnosis, screening and prevention
The most common symptoms of pneumonia are cough, breathlessness, chest pain, sputum production and fatigue 171 , 172 . Symptoms are not a part of the initial severity assessment of patients, as the initial symptom burden does not influence outcome. Exceptions include delirium, which is associated with an increased risk of mortality 173 , and pleuritic chest pain, which is associated with an increased risk of para-pneumonic effusion and complicated (infected) para-pneumonic effusion 174 , 175 . Usually mild disease refers to patients with CAP who do not require hospitalization, moderate disease to those cared for in conventional hospital wards, and severe disease to those admitted to the ICU.
It is not possible to differentiate bacterial and viral pneumonia based on symptoms in adults or children, as patients report similar symptoms regardless of microbial aetiology 176 . A recent study found that artificial intelligence was also unable to differentiate microbial aetiology based on symptoms, clinical features and radiology 177 .
CAP is usually clinically suspected in the presence of acute (≤7 days) symptoms of LRTI, such as cough, expectoration, fever and dyspnoea, as well as the presence of new infiltrates on chest radiographs (CXRs) 178 . In older patients, symptoms are typically less evident, and fever can be absent in as many as 30% of patients 179 . Symptoms may also be less evident in patients treated with steroids, NSAIDs and antibiotics 6 . Other pulmonary diseases — most frequently pulmonary embolism and lung cancer — may present with fever and pulmonary infiltrates that can mimic CAP. Interstitial and systemic diseases can also mimic CAP. When diagnosing CAP, it is extremely important to review prior chest CXRs if available, as an additional means to help rule out the disease.
Although HAP is also suspected clinically, symptoms may be hidden by either other medications or the cause of admission. No studies exist about symptom duration in HAP before diagnosis; however, it is usually suspected when patients present with pyrexia (fever) and/or tachypnoea (rapid breathing). HAP diagnosis is believed to be usually delayed, which could explain the higher mortality observed in this population than in patients with VAP.
VAP is suspected when there are at least two of the following symptoms: fever or hypothermia, leukocytosis or leukopenia, and evidence of purulent secretions in an endotracheal tube or tracheostomy 4 . For VAP diagnosis, clinicians often rely on clinical parameters; radiological and laboratory parameters help initiate antimicrobial treatment. Scores have been proposed to facilitate diagnosis. For example, the clinical pulmonary infection score (CPIS) 180 is the most common one, and it is based on points assigned to various signs and symptoms of pneumonia. A CPIS score of >6 suggests VAP, although score sensitivity and specificity are not perfect. In fact, the FDA does not accept this score to diagnose VAP in randomized controlled trials studying antibiotics. In patients with VAP, fever and pulmonary infiltrates can present as atelectasis (collapse of parts of the lung), alveolar haemorrhage and pulmonary thromboembolism, amongst other conditions. In a landmark study using immediate post mortem lung histopathology and microbiology as a gold standard, the presence of two clinical criteria plus the presence of infiltrates on CXRs had a 70% sensitivity and 75% specificity in the diagnosis of VAP 181 .
Radiographic confirmation is essential for the diagnosis of pneumonia. CXRs provide important information about the site, extent and associated features of pneumonia (for example, the lobes involved and the presence of pleural effusion and cavitation) 5 (Fig. 6 ). CXRs have a sensitivity and specificity of 43.5% and 93%, respectively, for detecting pulmonary opacities 182 . In CAP, sensitivity and specificity of 66% and 77%, respectively, have been reported 183 using CT scans as the gold standard. The presence of either pleural fluid or multilobar pneumonia serve as indicators of severity 5 . In CAP, the development of pulmonary infiltrates that were not previously present on a simple posterior–anterior (PA) CXR is essential for CAP diagnosis. The standard CXR for CAP consists of a PA and lateral images; the use of lateral projection images increases diagnostic performance of PA images. In HAP, radiographic evidence of infiltrates is usually determined by CXR examination alone. In VAP, new infiltrates are usually detected by anterior–posterior projection in the supine position; however, in this situation, CXRs are insufficiently sensitive and specific.
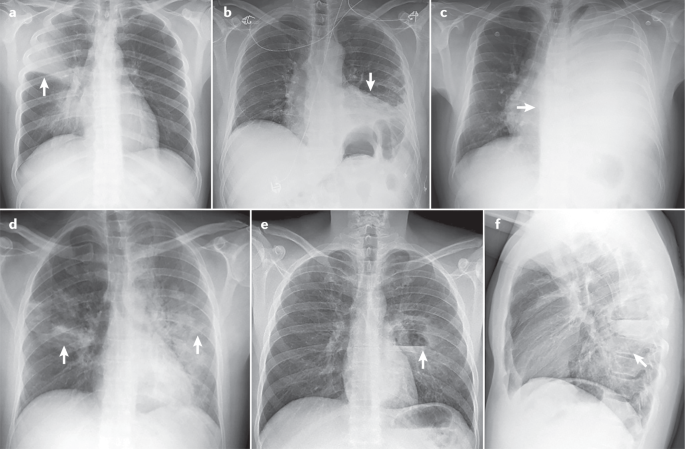
Pneumonia in upper right lobe (arrow) (part a ); pleural effusion on the left side (arrow) (part b ); massive pleural effusion in the left lung (arrow) (part c ); bilateral pneumonia (arrows) (part d ); lateral image showing left parahilar cavitation with air–fluid level in the lower left lobe (arrow) (part e ); front-to-back image in the same individual as in part e .
In studies in patients hospitalized with CAP, CT identified up to 35% of patients with CAP who had not initially been caught by CXRs 184 . In many patients with COVID-19, CT scans detect pulmonary infiltrates not observed on simple CXRs 185 . In patients with CAP, CT scans serve as a practical complement to CXRs in several cases: when radiographic findings are non-specific, when pulmonary complications such as empyema (pus in the pleural space) or cavitation are present, when there is suspicion of an underlying lesion such as lung carcinoma, and when recurrent pneumonia or non-resolving pneumonia is present 186 . Although this supporting role of CT scans is assumed to apply to patients with HAP as well, supporting evidence is lacking.
Ultrasonography
Lung ultrasonography is a non-invasive imaging method that is now frequently used in many emergency departments and ICUs. Advantages over CT include the absence of radiation exposure, ready use at the bedside and reasonable diagnostic sensitivity and specificity 187 . However, the technique has a steep learning curve, especially in mechanically ventilated patients. In a systematic review, lung ultrasonography was shown to have a sensitivity of 88% and a specificity of 89%, with a ~90% probability of diagnosing pneumonia 188 . Echographic diagnosis is more complex in patients with VAP, and only a few observational studies have been conducted to date 188 . The best of these studies have shown that such diagnosis had better accuracy than the CPIS score alone; the addition of direct Gram stain examination in quantitative cultures of endotracheal aspirates further improved accuracy 189 , 190 . On the basis of on these results, the ventilator-associated pneumonia lung ultrasound score (VPLUS) was developed, and has a sensitivity of 71% and a specificity of 69% for VAP diagnosis 190 .
Microbiology and laboratory tests
Recommendations for microbiological diagnosis in CAP vary according to disease severity (Table 2 ). Of note, microbiological diagnosis in CAP cannot be obtained in up to 50% of patients 5 . In patients with CAP who do not need hospital admission, obtaining samples such as sputum and pharyngeal swabs is optional or not recommended in recent guidelines 5 . In patients requiring hospitalization, obtaining good-quality sputum and blood samples, as well as pharyngeal swabs (for PCR), is recommended. Sputum is the most common respiratory sample in patients with CAP, and samples should be collected before antibiotic treatment. The sensitivity of Gram staining for a sputum sample is ~80% in patients with pneumococcal pneumonia and 78% in patients with pneumonia caused by Staphylococcus spp., and the specificity is 93–96% 191 , 192 . Most health care institutions perform viral PCR on pharyngeal swabs during the influenza season. In the COVID-19 pandemic, it is recommended that all patients admitted with CAP receive a PCR test for the detection of SARS-COV-2.
In patients requiring ICU admission, in addition to all tests mentioned above, bronchoscopic samples, such as bronchoalveolar lavage (BAL) in intubated patients, are not difficult to obtain and provide information on the lower respiratory tract microbiota. Urinary antigen detection tests for S. pneumoniae and L. pneumophila have good sensitivity and specificity, are not extremely expensive and are recommended in all hospitalized patients.
In patients with HAP or VAP, international guidelines 4 recommend obtaining distal respiratory samples for semiquantitative or quantitative cultures (Table 3 ). In patients with HAP, bronchoscopy is not easy to perform, and sputum samples are not often collected. In patients with VAP, distal respiratory samples are preferred. BAL (performed with or without concomitant bronchoscopy) is the sample that provides most information, as, in addition to cultures, cellularity analysis and PCR can be performed on the fluid. A recent meta-analysis showed that Gram staining of BAL performs well in detecting S. aureus 193 . Respiratory samples from patients with HAP or VAP have to be collected before the initiation of a new antibiotic treatment to avoid false-negative cultures. International guidelines 4 do not recommend using procalcitonin (PCT) for the initial diagnosis of HAP or VAP, as several studies have shown that it lacks diagnostic value 194 .
Since the 2000s, owing to multiple outbreaks, epidemics and pandemics caused by respiratory viruses in particular, several molecular tests have been developed, which have contributed to widened availability of molecular testing for the aetiological diagnosis of CAP. Molecular tests have several advantages, including detecting low levels of microbial genetic material, remaining unaffected by prior antibiotic therapy, and providing results within a clinically relevant time frame 195 . Molecular tests based on multiplex PCR have been developed to simultaneously detect and quantify multiple respiratory pathogens, as well as some genes related to antimicrobial resistance. Several commercial multiplex platforms are currently available for comprehensive molecular testing for respiratory pathogens that cause pneumonia (respiratory viruses, bacteria and fungi) and for the main resistance genes of the most common bacteria causing pneumonia 195 , 196 , 197 , 198 .
The WHO currently recommends COVID-19 diagnosis by molecular tests that detect SARS-CoV-2 RNA. SARS-CoV-2 viral sequences can be detected by real-time reverse transcriptase (RT-PCR) in nasopharyngeal swab samples 199 . The disadvantage of this method is that it requires specialized equipment and trained personnel. Additionally, two types of rapid tests are available for COVID-19 diagnosis. The direct SARS-CoV-2 antigen test detects viral components present during infection in samples such as nasopharyngeal secretions, and, therefore, can indicate whether an individual is currently carrying the virus. The indirect antibody test detects antibodies that can be found in serum as part of the immune response against the SARS-CoV-2; thus, it can yield false-negative results if performed before the antibody response has developed and cannot distinguish between past and current infections. These two tests are relatively simple to perform and interpret, requiring limited test operator training 199 .
Some biomarkers may be helpful in identifying which patients are likely to have bacterial pneumonia, in deciding whether antibiotic therapy should be administered, in determining prognosis and in facilitating decisions related to the site of care. However, biomarkers should only be used as an adjunctive tool when managing CAP, as no biomarker has proven full utility in predicting clinical outcomes in patients.
The most widely used biomarkers are acute phase reactants such as C-reactive protein (CRP) and PCT 200 . However, their serum kinetics differ: CRP levels increase after the first 3 days of infection (peak time from infection is 36–50 h), whereas PCT levels rise rapidly (peak time from infection is 12–24 h) in response to microbial toxins or host responses. These properties are useful in differentiating CAP from other non-infectious causes. CRP levels increase in response to any inflammation, and can be modified by the presence of corticosteroids and previous antibiotic therapy, whereas PCT is more specific in bacterial pneumonia. Viral infection-related cytokines attenuate induction of CRP and PCT; however, some elevation in their levels can occur when pneumonia is caused by atypical pathogens (for example, Mycoplasma spp., Chlamydia spp. and Legionella spp.) 201 .
Both CRP and PCT can assist in the clinical diagnosis of pneumonia, but CRP and PCT cannot be used in isolation as a basis for treatment decisions. A second test after 24–48 h is mandatory to monitor for any increases. Clinicians should also consider the pattern in the days preceding symptom onset in patients with CAP and whether a patient is taking medication that could have modified these values. For patients with radiographic CAP, PCT levels can be used with clinical assessment to identify those individuals from whom antibiotic therapy can be safely withheld. This assessment can be combined with a PCR test to identify viral infection, especially as new data show that viruses can frequently be a cause of CAP 13 , 75 . However, caution should be used when a mixed viral–bacterial infection is considered. The new American Thoracic Society (ATS)/Infectious Diseases Society of America (IDSA) CAP 5 guidelines do not recommend using PCT to determine the need for initial antibacterial therapy. The current recommendation is that empirical antibiotic therapy should be initiated in adults with clinically suspected and radiographically confirmed CAP, regardless of initial PCT level.
In studies in patients with HAP or VAP, in whom biomarkers had been monitored serially since before infection, steady increases or persistent elevations in CRP levels were shown to be associated with a high risk of VAP 202 . However, no such pattern was shown for PCT values (crude values or kinetics), with poor diagnostic accuracy for VAP 203 . Thus, a recent international consensus concluded that a combination of clinical assessment including PCT levels in well-defined antibiotic stewardship algorithms could improve diagnosis of bacterial infections and support antibiotic effectiveness 204 .
Prevention of CAP
Many factors increase the risk of CAP and can generally be divided into host factors (for example, age, and the presence of COPD and other chronic pulmonary diseases, diabetes mellitus and chronic heart failure), unhealthy habits (for example, smoking and excessive alcohol consumption) and medications (for example, immunosuppressive drugs, sedating medications such as opioids, and proton pump inhibitors within the first 3 months of administration 205 ). Prevention of CAP is crucial, especially in individuals with these risk factors. Available preventive measures include smoking and alcohol use cessation, improvements in dental hygiene, physical exercise, avoiding contact with children with respiratory infections, and pneumococcal and influenza vaccinations 14 . Implementing these measures in primary and specialized care could help reduce the burden of CAP. Presently, pneumococcal and influenza vaccination are the cornerstones of CAP prevention.
The 23-valent pneumococcal polysaccharide vaccine (PPV23) and the 13-valent pneumococcal conjugate vaccine (PCV13) are currently used in adults. Owing to the demonstrated effectiveness of PPV23 in preventing invasive pneumococcal disease (IPD) in people of ≥65 years of age, the use of the vaccine in this population is recommended in many countries 206 . However, PPV23 effectiveness in preventing non-IPD or CAP due to any cause is much less clear. The effectiveness of PPV23 has been reported to range from 25% to 63% in pneumococcal pneumonia 207 , 208 ; the effectiveness of PCV13 in preventing the first episode of CAP, non-bacteraemic and non-invasive CAP, and IPD due to serotypes contained in the vaccine amongst adults of ≥ 65 years of age has been reported to be 45.6%, 45% and 75%, respectively 209 . Efficacy persisted through the mean follow-up period of 4 years 209 . A post-hoc analysis based on data from the CAPITA trial showed that the effectiveness of PCV13 ranged from 43% to 50.0% for pneumococcal CAP, 36% to 49% for non-bacteraemic and non-invasive pneumococcal CAP, and 67% to 75% for pneumococcal IPD 210 . Of note, the most important measure in reducing pneumococcal CAP burden (bacteraemic and non-bacteraemic) in adults is conjugate vaccine programmes in children. Vaccination with pneumococcal conjugate vaccine in children substantially reduces disease in adults owing to the interruption of transmission and herd protection 211 , 212 .
Influenza vaccination can reduce the risk of complications of influenza, such as pneumonia, and is associated with a decrease in severity, hospitalization, ICU admission and mortality associated with influenza 213 , 214 . All age groups can be affected by influenza virus infection; however, older individuals, young children, pregnant women and those with underlying medical conditions have the highest risk of severe complications. In 2019, a study 75 found that viral sepsis was present in 19% of patients with CAP admitted to ICU and in 61% of patients with viral CAP; influenza virus was the main aetiology. More recently, a study 215 found influenza virus in 23% of patients with LRTI; 57% of these patients had radiographically confirmed CAP. The authors reported 35% vaccine effectiveness against influenza virus LRTI and 51% against influenza-associated CAP. These data demonstrate the importance of an annual influenza vaccination, especially in at-risk groups.
Prevention of HAP
HAP is the leading cause of death from hospital-acquired infection; however, only limited effort has been made in developing prevention strategies. HAP occurs owing to pharyngeal colonization with pathogenic organisms and, in the case of VAP, subsequent aspiration. Thus, oral care and precautions against aspiration may attenuate some of the risk. Although oral and/or digestive decontamination with antibiotics may also be effective, this approach could increase the risk of selecting resistant organisms. Other preventive measures, including isolation practices, remain theoretical or experimental. Indeed, most potential prevention strategies for HAP remain unproven 216 .
The individual measures included in prevention bundles can be divided into non-pharmacological and pharmacological categories. To date, most of our knowledge in HAP prevention is extrapolated from prevention strategies for VAP. An important concept in these strategies is that no single measure is deemed adequate to ensure prevention, with prevention bundles advocated instead. A prospective, interventional, multicentre study in Spain, the Pneumonia Zero project 217 , which included 181 ICUs and built on the experience from a previous study 218 , suggested VAP prevention via a bundle of mandatory and highly recommended measures. The mandatory measures were education and training of medical staff in airway management, hand hygiene with alcohol solutions, oral hygiene with an antiseptic (chlorhexidine), semirecumbent positioning and promotion of procedures and protocols that safely avoid or reduce duration of mechanical ventilation. The highly recommended measures were aspiration of subglottic secretions (removal of secretions that accumulate above the endotracheal tube cuff, in patients who were expected to be mechanically ventilated for >72 h), selective digestive decontamination (SDD)), and selective oropharyngeal decontamination (SOD) (prophylactic strategies to prevent or minimize infections in critically ill patients, based on the application of non-absorbable antibiotics in the oropharynx and gastrointestinal tract (SDD) or oropharynx (SOD) of patients). When implemented, these measures enabled a decrease in adjusted frequency of VAP from 9.83 to 4.34 per 1,000 ventilator-days over 21 months; similarly, the percentage of patients with VAP significantly decreased from 2.4% to 1.9%. In the ICUs with prolonged participation in the study (19–21 months), the incidence of VAP significantly decreased further to just 1.2%. Finally, significant decreases were observed in VAP recurrence rates (from 10.9% to 7.7%).
Non-pharmacological measures
Good hand hygiene using alcohol solution before airway management is firmly established as a fundamental component of clinical practice. Its inclusion in the VAP care bundle represents an opportunity to audit compliance with, and optimize the quality of, hand hygiene practices 217 , 219 .
Remaining in the supine position 220 , the use of gastric tubes and the presence of contents in the stomach contribute to the reflux of gastric contents, aspiration and VAP. Semirecumbent positioning at 30–60° may help to avoid these problems, as found in a 2016 meta-analysis 221 . The lateral Trendelenburg body position (the patient is positioned inclined with head down and feet elevated) has shown no substantial benefit, with research even showing an increase in the number of adverse events 222 . However, based on the results of a post-hoc analysis of the Gravity VAP trial, patients without pulmonary infiltrates at intubation and with no contraindications for the approach may benefit from this position for a short period 222 . The prone position is used to improve hypoxaemia in patients with severe ARDS 223 . This measure is frequently used in COVID-19-associated ARDS 224 , 225 . This approach might decrease the incidence of VAP, as it facilitates the drainage of secretions compared with a semirecumbent position 226 . Further confirmation is needed to assess the beneficial effect in reducing VAP in patients with COVID-19.
Endotracheal tubes also have an important role in the pathogenesis of VAP, and removing contaminated oropharyngeal secretions can reduce the risk of VAP. In a meta-analysis from 2016, evidence supported the use of endotracheal tubes with subglottic secretion drainage to decrease the rate of VAP 227 . Maintaining cuff pressure at >25 cmH 2 O may further prevent the leakage of bacterial pathogens into the lower respiratory tract 217 , and continuous cuff pressure regulation could be superior to intermittent control for preventing VAP 228 . Finally, the tube cuff shape and material may have a role in the aspiration of secretions; a randomized, multicentre trial showed that cuffs made of polyurethane or of a conical shape were not superior to conventional cylindrical polyvinyl chloride cuffs in preventing tracheal colonization and VAP 229 .
Pharmacological measures
Oral washing with chlorhexidine seems to be effective in preventing VAP; however, a recent meta-analysis 230 showed a trend for increased mortality in patients who received chlorhexidine. Consequently, recent international guidelines 3 did not recommend its use. It is plausible that this increased mortality could be due to direct lung toxicity from aspirated chlorhexidine.
Furthermore, the use of either SOD or SDD remains controversial, with most studies to date being performed in settings with low prevalence rates of MDR or XDR microorganisms. These studies have shown a decrease in both the incidence of VAP and overall mortality 231 . However, in a recent cluster randomized clinical trial performed in units with high rates of MDR or XDR pathogens, SOD and SDD were not effective in decreasing bacteraemia caused by those microorganisms 232 . SDD and SOD are not applied in many centres in the USA and in Europe, primarily for fear of inducing microbial resistance. Owing to the unclear balance between a potential reduction in pneumonia rate and a potential increase in mortality, the 2017 international guidelines 3 decided not to issue a recommendation on the use of chlorhexidine for SOD in patients requiring mechanical ventilation until more safety data becomes available. However, the guidelines did suggest the use of SOD — but not SDD — in settings with low rates of antibiotic-resistant bacteria and low antibiotic consumption. Although establishing a cut-off value for low and high resistance settings is a dilemma, the committee felt that a 5% threshold was reasonable.
Prevention of recurrent pneumonia
Recurrent pneumonia affects ~9% of patients hospitalized with CAP 233 , 234 . The main factors related to recurrent pneumonia are age ≥65 years, lack of pneumococcal vaccination, previous episode of pneumonia, COPD and corticoid therapy. S. pneumoniae is the most frequently identified pathogen in patients with recurrent pneumonia 233 , 234 . The main preventive measures for recurrent pneumonia are vaccination and adequate control of prior comorbidities, especially in an older population who have an increased risk of infection.
Antibiotics are the mainstay of therapy for pneumonia; however, the agents used depend on a variety of host and pathogen factors. Ideally, therapy should be pathogen-directed, even though a pathogen is often not identified. Nevertheless, as therapy must be started promptly, empirical therapy directed at the most likely aetiological pathogens is required. Because empirical therapy may be more broad-spectrum than definitive therapy, it is often necessary to narrow and target antibiotics once diagnostic testing results become available, usually after 48–72 h. Such a strategy is referred to as a ‘de-escalation’ of therapy 235 . Rapid comprehensive multiplex molecular methods have been cleared by the FDA and provide results within 2–4.5 h, prior to obtaining final diagnostic testing data. These methods include antibiotic resistance markers and facilitate identification of specific viruses and bacteria, thereby aiding in therapeutic choices and the escalation, de-escalation or cessation of antibiotics.
Considerations for therapeutic choices
Relevant host factors for choosing the type of empirical therapy are severity of illness, the presence of specific medical comorbidities and certain historical data. In detail, these include: chronic lung, heart or liver disease; diabetes mellitus; asplenia; alcohol use disorder; malignancy; malnutrition; recent hospitalization, antibiotic use or colonization by drug-resistant bacteria; the presence of risk factors for aspiration of gastric contents into the lungs (such as impaired swallowing, vomiting, altered consciousness and impaired cough reflex); and recent contact with a health care environment (for example, patients requiring haemodialysis) 236 . It is also important to know epidemiological data regarding individual patients. Seasonal viruses such as influenza viruses are worth examining during the autumn and winter. Contact with someone known to have an illness transmitted by an airborne route (for example, tuberculosis) is also relevant. Similarly, residence in an area with endemic mycoses is a risk for certain fungal pneumonias. Finally, an ICU with a high rate of drug-resistant pathogens poses a risk factor for VAP caused by such organisms 3 .
The site of pneumonia acquisition is also an important consideration, namely, in the community, hospital or ICU, or whilst on mechanical ventilation. Since the late 1990s, guidelines have been developed for patients with pneumonia in each of these settings; however, recent data suggest that patient risk factors, and not the site of infection, should be the main determinant for empirical antibiotic choice. Recently, a unified algorithm based on these risk factors has been proposed for all patients with pneumonia 236 .
In addition to choosing an antibiotic that is likely to target the aetiological pathogens, it is equally important to determine the right dose and route of administration, to ensure that the drug penetrates into the site of infection. In general, oral therapy is used in patients with less severe illness, whilst intravenous therapy is administered in patients with more serious illness. Aerosolized therapy can be used to boost drug delivery to infected lung tissue, especially if the chosen drugs penetrate into the lung poorly. When treating a critically ill patient with pneumonia and a MDR pathogen, it may be necessary to use high doses to ensure reaching bactericidal drug concentrations at the site of infection. Continuous or prolonged infusion may be needed in the case of β-lactam antibiotics to maximize the time during which the drug concentration exceeds the minimum inhibitory concentration (MIC) of the target organism. Other drugs, such as aminoglycosides, kill bacteria in a concentration-dependent fashion and are best administered at high dosages given once daily 237 . In young patients with pneumonia and sepsis, drug clearance by the kidney may be accelerated (augmented renal clearance), and dosing will need to be increased appropriately 238 . In those with renal impairment, dosing or the frequency of administration may need to be reduced and can be optimized by therapeutic drug monitoring, if available.
CAP therapy
Guidelines for CAP recommend empirical therapy based on the severity of illness and presence of risk factors for specific complex pathogens 5 , 53 , 239 (Table 4 ). In the past, patients with risk factors that included contact with a health care environment (haemodialysis, recent hospitalization, residence in a nursing home) were considered to have HCAP and were treated differently from patients with CAP. The new guidelines have eliminated HCAP as a category and recommended that these patients be treated as having CAP. Without forgoing consideration of the local frequency of penicillin and macrolide resistance, every patient with CAP should be treated for pneumococcus in most parts of the world. In addition, atypical pathogens may have a role, often as co-infecting agents; studies showed improved patient outcomes when macrolides or quinolones were added to β-lactam therapy in patients with CAP, particularly those with more severe illness 240 , suggesting a need to treat atypical pathogens in many patients with CAP. Patients with more severe illness may need empirical therapy for MRSA and/or P. aeruginosa , especially if colonization had occurred previously following influenza (in the case of MRSA) or after prior use of broad-spectrum antibiotics (for both pathogens) 241 .
Although in many patients CAP may have a viral aetiology, either as a single pathogen or as part of a mixed infection, antiviral therapy is not routinely recommended. However, for documented influenza-associated pneumonia, current guidelines recommend the use of an anti-influenza agent such as oseltamivir, regardless of illness duration 5 . Nonetheless, the benefit of these agents is greatest within the first 48 h of infection onset. Thus, in patients with a high suspicion of influenza, therapy should be started, whilst results from diagnostic testing are pending. Additionally, even with documented influenza, antibiotics should be used empirically to account for possible bacterial superinfection 5 .
Outpatients
For outpatients without comorbidities or risk factors for MDR pathogen infection, current guidelines recommend monotherapy with respiratory fluroquinolone or combination with amoxicillin–clavulanate or a cephalosporin and macrolide or doxycycline 5 . Regardless of the prevalence of resistance, good experience with macrolide monotherapy has been reported, suggesting that in vitro resistance is not always clinically relevant unless it is high-level resistance (resulting from a ribosomal mechanism) and not lower-level resistance (caused by efflux pumps) 242 . For example, in a Canadian study, patients with CAP who received macrolide therapy (usually as monotherapy) had lower mortality and hospitalization rates than those receiving alternative therapies 243 . For outpatients with comorbid illnesses, current guidelines recommend therapy with a β-lactam and macrolide combination or monotherapy with a respiratory fluoroquinolone, even though recent concerns about fluoroquinolone toxicity have limited their use 5 .
Hospitalized patients
In patients with CAP in hospital wards, therapy should be a β-lactam–macrolide combination or a quinolone (levofloxacin or moxifloxacin) alone (Table 4 ). In areas with a high prevalence of endemic tuberculosis, caution should be exercised with the use of a quinolone, as it can mask the presence of tuberculosis and select for drug-resistant tuberculosis. β-Lactams include ceftriaxone, ceftaroline and ampicillin–sulbactam, whilst macrolides should comprise azithromycin or clarithromycin; some recent data have shown more frequent cardiac complications with the use of erythromycin 244 . Many studies have shown that the addition of a macrolide to the β-lactam, particularly in those with moderately severe illness or with Legionella spp. infection, is associated with a lower mortality rate than β-lactam monotherapy 245 .
All ICU-admitted patients should receive a combination therapy of a β-lactam and either a macrolide or a quinolone. Admission to ICU should be guided by the presence of one of two major criteria (need for mechanical ventilation or septic shock requiring vasopressors) or three of nine minor criteria, as per the 2007 ATS/IDSA guidelines 239 . In this population, a macrolide is generally preferred, although some studies have shown that a quinolone may prove more effective if Legionella spp. infection is highly suspected or documented 246 . If the patient has risk factors for P. aeruginosa or MRSA infection, then treatment for such pathogens should be added.
HAP therapy
Patients can develop HAP in or outside the ICU and can be managed with or without mechanical ventilation, although as many as 30% of patients with HAP who are not initially ventilated will require mechanical ventilation 247 . In patients with a predicted mortality risk of <15% based on the presence or absence of septic shock, monotherapy is associated with lower mortality than combination therapy. In patients with a predicted mortality risk of >25%, combination therapy is associated with reduced mortality; the type of therapy has no effect on mortality in those with a predicted mortality risk of 15–25% 248 . MDR pathogen infection should be considered in patients with a history of prior antibiotic therapy or prolonged hospitalization in the previous 3 months, as well as patients hospitalized in an ICU with a >25% rate of MDR pathogen infections. Although empirical therapy can be guided by patient features, each ICU has its own unique bacteriology; thus, therapy should be guided by knowledge of the local antibiogram 3 , 249 .
Patients with a low mortality risk (estimated from published data in relation to the presence of sepsis and shock) and no MDR pathogen risk factors should receive monotherapy (Table 5 ). In patients with a mortality risk of >15% and/or risk factors for MDR pathogens but who are not in septic shock, monotherapy can be adequate (provided that the chosen antibiotic can target >90% of the gram-negative pathogens in the ICU). Although there is controversy in many hospitals about the need for combination therapy, two agents are often necessary to provide a >90% likelihood of appropriate therapy, especially in the high-risk population and in those with septic shock. The combination regimen should target P. aeruginosa and ESBL-producing Enterobacterales. In all patients with HAP, anti-MRSA therapy should be considered and, if necessary, administered with either vancomycin or linezolid. Depending on local epidemiology, some patients will be at risk of infection with Acinetobacter baumanii , carbapenem-producing Enterobacterales or Stenotrophomonas maltophilia , each one requiring a unique therapy approach. For VAP due to MDR pathogens, such as Acinetobacter baumanii , adjunctive inhaled antibiotics (amikacin or colistin) have been added to systemic therapy, with no proven mortality benefit; efficacy may vary with the type of aerosol delivery system used 250 .
The duration of HAP therapy is between 7 and 14 days, although most patients are successfully treated within only 7 days 251 . Although not all experts agree, the European guidelines list the following groups as exceptions to short duration therapy: patients with MDR pathogen infection, such as P. aeruginosa and Acinetobacte r spp.; those who received inappropriate therapy initially; those who are severely immunocompromised; and those receiving second-line antibiotic agents 217 , 252 , 253 . Current guidelines do not strongly endorse biomarkers such as PCT to guide therapy duration for HAP and VAP, although some randomized trial data do show efficacy for this approach 254 .
Therapy in immunocompromised patients
Immunocompromised patients can develop pneumonia due to the common community and nosocomial pathogens present in the setting as well as other pathogens related to a specific type of immune dysfunction and/or resistant bacteria, viruses, fungi and parasites. Common conditions that impair the immune system include malignancy, HIV infection with a CD4 + T cell count of <200 cells per mm 3 , and solid organ or stem cell transplantation. Therapies that cause immune suppression include prednisone, biological disease modifiers, and chemotherapeutic agents such as azathioprine, methotrexate and cyclophosphamide.
Although empirical therapy is often used, the range of possible pathogens in this population is so broad that aggressive diagnostic testing is necessary, including sampling of deep lower respiratory tract secretions with bronchoscopy in most patients 255 . In patients with HIV infection and a low CD4 + T cell count or with recent corticosteroid tapering, therapy should target common pathogens and Pneumocystis jirovecii 256 . Patients with severe neutropenia, steroid-induced immune suppression and those receiving biologic response modifiers (such as tumour necrosis factor inhibitors) can be infected with fungi such as Aspergillus spp. or Mucorales. Diagnostic testing in those with malignancy or drug-induced immune suppression should also consider other opportunistic pathogens, including cytomegalovirus, Varicella zoster virus, Nocardia spp., parasites such as Strongyloides stercoralis and Toxoplasma gondii , and Mycobacterium tuberculosis (for example, owing to a re-emergence of latent infection).
Aspiration pneumonia therapy
Patients with witnessed macro-aspiration of gastric or oral contents into the lung can develop chemical or bacterial pneumonitis, or simply have bland aspiration. If bacterial pneumonia occurs, patients should receive antibiotics aimed at common community or nosocomial pathogens that were likely to be colonizing the oral and gastric tract at the time of aspiration. In community aspiration, therapy is the same as in CAP unless the patient has poor dentition, which can make infection by anaerobic pathogens possible owing to favourable growth conditions for such microbes in the patient’s mouth. When patients with poor dentition have a lung infiltrate after a witnessed or clinically suspected aspiration event, therapy should be a β-lactam such as ampicillin–sulbactam or amoxicillin–clavulanate, or a quinolone, such as levofloxacin or moxifloxacin. Any of these drugs could also be used if dentition is normal; alternatively, ceftriaxone would be effective 1 . For those with nosocomial aspiration, therapy should be based on the presence of risk factors for MDR pathogens and aimed at common, local and drug-resistant organisms, similar to therapy in other forms of nosocomial pneumonia. There is no need to add specific anti-anaerobic coverage, as these organisms are uncommon in patients who aspirate whilst in hospital or chronic care facilities 257 .
Adjunctive therapy
In addition to antibiotics, patients with severe illness might benefit from adjunctive corticosteroid therapy. In general, this therapy should be restricted to those with severe CAP and a high inflammatory response 258 . In one trial, methylprednisolone was more effective than placebo, leading to less treatment failure (especially late failure) in a population with both severe CAP and elevated CRP levels in the serum 259 . However, before using corticosteroids, it is necessary to rule out influenza, as it may worsen with this line of therapy 260 . By contrast, studies in patients with COVID-19 and hypoxaemic respiratory failure have shown a benefit of corticosteroid therapy with dexamethasone 261 . Similarly, IgM-enriched immunoglobulin may be useful in patients with severe CAP, and high CRP levels and low IgM levels in the serum. In a randomized, double-blind, placebo-controlled trial, IgM-enriched immunoglobulin led to a reduction in mortality and an increase in ventilator-free days in this population, when compared with placebo 262 .
Another adjunctive and supportive therapy includes management of hypoxaemia with respiratory failure, which may necessitate mechanical ventilator support. However, some studies show that patients with CAP can be managed with either non-invasive ventilation or high-flow oxygen. Either modality can reduce the need for mechanical ventilation and, therefore, avoid some of the complications associated with endotracheal intubation and ventilation 263 .
Follow-up of patients after pneumonia
In some patients with CAP, pneumonia can be the start of an inexorable downhill course. In one study, the long-term mortality of patients of >65 years of age hospitalized with CAP far exceeded the in-hospital mortality (33.6% and 11%, respectively) 264 . In some studies, this long-term effect has been attributed to cardiac events that were initiated by acute lung infection 155 .
Pneumonia recurrence can occur in all forms of pneumonia. Recurrence should be classified on the basis of the site of infection. If re-infection occurs at the same site as the original infection, consideration should be given to local factors such as endobronchial obstruction (due to a tumour or foreign body), focal bronchiectasis, insufficient duration of therapy, or infection with a drug-resistant or inadequately treated pathogen. Recurrence elsewhere could be due to immune impairment (due to comorbid illness or certain medications), a non-infectious pulmonary process or recurrent aspiration.
Routine follow-up chest radiography after CAP is not generally recommended. However, if it is prescribed (to monitor resolution of a pleural effusion or infiltrate suggestive of a possible lung mass), it should be delayed for 4–6 weeks if the patient is responding well to therapy 5 . During follow-up, patients should be monitored for undiagnosed or ineffectively managed comorbid illness and encouraged to avoid cigarette smoking. Patients should also have up-to-date pneumococcal and influenza vaccinations. The 30-day readmission rate for patients with CAP has been found to vary from 16.8% to 20.1% 167 . Pneumonia itself was the cause of readmission in only 17.9–29.4% of patients; however, other common causes were exacerbations of congestive heart failure or COPD 167 . Patients with health-care-associated risk factors have a higher probability of readmission than patients with uncomplicated CAP 265 .
Quality of life
The effect of pneumonia is heavily influenced by both the origin of the disease (within the community or in health care environments) and its severity 266 . Most data regarding the effect on quality of life have been obtained in patients with CAP 171 . Antibiotic treatment starts to improve pneumonia symptoms rapidly; acute symptoms typically improve within 3–5 days in patients with mild CAP (outpatients) and 5–10 days in hospitalized patients with more severe CAP not requiring ICU admission; however, return to baseline levels of symptoms and function seems to take substantially longer 172 , 267 , 268 , 269 . In mild-to-moderate CAP, in most patients symptoms such as cough and breathlessness resolve within 14 days, although up to 6 months are required for full recovery 267 . Thus, the greatest burden seems to be a loss of function in the long term. Delayed recovery is associated with the number of comorbid conditions. In most cases, the presence of ongoing health impairment is largely related to a decompensation of underlying diseases rather than the ongoing acute symptoms of CAP 267 . A modelling study showed that in hospitalized patients with CAP, these acute symptoms reduced in intensity by ~50% within the first 3–5 days, and resolved in nearly all patients by day 28 (ref. 268 ). There does not seem to be a meaningful difference in symptom intensity or time to symptom resolution between viral and bacterial pneumonia 270 .
A French study in patients with pneumococcal pneumonia followed for 12 months after hospital discharge used the EQ-5D-3L questionnaire to evaluate health status 271 . Patients experienced a progressive improvement in quality of life after discharge, plateauing at six months. Importantly, quality of life either did not improve or deteriorated after discharge in 34% of patients; recovery was worse in old patients than in young patients. In a US study in patients with CAP, on average, patients were able to return to normal productivity in 3 weeks and missed 2 weeks of work 272 . Recovery was slowest in patients with comorbidities such as COPD, leading to recovery times of 2 months on average. Even after recovery, symptom scores in patients with CAP are worse than those in the general population, partially because CAP has a long-term effect on health. Another partial reason for these lower scores is the development of CAP in patients with high-risk comorbidities, which make these patients more symptomatic than the general population 273 . Lastly, long-term mortality is increased in patients with CAP compared with the general population 35 . LRTIs without radiographic infiltrates (non-CAP LRTIs) are associated with a similar impairment in quality of life to CAP 274 .
Studies comparing quality of life between patients with CAP and the general population have shown consistently worse quality of life up to 12 months after CAP. With a few exceptions, most of these studies used generic quality of life and productivity tools. A systematic review identified five CAP-specific, patient-reported outcome measures, of which the CAP symptom questionnaire (CAP-sym) was the most widely used 275 . This review concluded that most CAP-specific tools have thus far been evaluated in highly specific populations and may not be fully representative, and it recommends continuing to use generic tools until better tools are available.
Improved diagnostics
The key to a switch to pathogen-specific therapy is an accurate aetiological diagnosis, and the availability of rapid molecular diagnostic tests makes clinical trials and subsequent clinical use of these targeted therapies feasible. Most progress in diagnostics can be observed in two areas: rapid identification of pathogens in positive blood cultures and detection of respiratory viral pathogens. However, bacteraemia is uncommon in pneumonia and, therefore, the effect of these molecular assays on management is limited. By contrast, PCR diagnosis of respiratory viral infections has now become the standard of care. The greatest issue with these assays obtained from nasopharyngeal specimens is whether results reflect upper respiratory tract infections only or accurately detect the cause of pneumonia. In addition, negative nasopharyngeal samples have occurred in patients with positive concurrent bronchoalveolar samples for influenza and SARS-CoV-2 (ref. 276 ).
Several multiplex PCR platforms are available for clinical use for bacterial pneumonia, with approval based on comparison with standard diagnostic tools, specifically culture 277 , 278 . However, as culture itself is not a gold standard, the true operating characteristics of the tests remain unknown. One alternative is metagenomics sequencing to determine all microbiota present; clinically relevant platforms are available 279 , 280 . Generally, these molecular assays are more sensitive than culture, especially for fastidious microorganisms; nevertheless, none of the current multiplex assays detect all of the relevant pathogens and, therefore, cannot replace cultures. In addition, a limited ability to provide information on antibiotic susceptibility is a major weakness. Despite such limitations, substantial impact on antibiotic prescription is possible. Most evaluations to date comprise observational studies and analyses of the theoretical benefit if antibiotic decisions based on molecular assays were applied prospectively. Perhaps the best demonstration of such potential is to limit the use of vancomycin or linezolid for suspected MRSA pneumonia 197 . Multiple sensitive and specific gene targets for S. aureus identification are available, whilst the absence of the mecA gene detection essentially excludes methicillin resistance in that isolate; thus, a negative assay eliminates the need for MRSA coverage. However, the greatest hurdle for molecular assays is clinicians’ willingness to base antimicrobial treatment on results obtained from these novel diagnostic platforms; even a BAL assay with a 98% negative predictive value did not result in a decrease in empirical treatment of VAP 281 . Implementation trials are required to demonstrate the true benefit of more accurate diagnostics.
Improved diagnostic testing may enable a host of unanswered epidemiological matters surrounding pneumonia to be addressed. A leading question in the field of pneumonia is its cause in immunocompromised patients; only expert opinion guides treatment recommendations 256 . The COVID-19 pandemic also illustrates the probable high frequency of additional viral agents that may cause CAP of seemingly unknown aetiology 13 . The role of fungal superinfection of viral pneumonia also remains controversial owing to diagnostic uncertainty 282 .
Antibiotic therapy
For most of the ~75-year history of antibiotic treatment of pneumonia, the backbone of therapy has been a β-lactam 283 . The emergence of bacterial resistance to β-lactams has been tackled with two strategies: newer generations or types of β-lactams (penicillins, cephalosporins and carbapenems) 284 , 285 , 286 , 287 , 288 and combinations with β-lactamase inhibitors (BLIs). Ceftolozane is the newest β-lactam on the market; it has improved activity against P. aeruginosa compared with other cephalosporins 284 . Each BLI has slightly different activity against the variety of resistance mechanisms in Enterobacterales, including carbapenem-resistant and ESBL-producing Enterobacterales, which may affect local efficacy owing to geographical differences in resistance patterns 289 .
Each new drug had been intended to replace the prior generation, gain a large proportion of market share and, therefore, justify the large development costs for the pharmaceutical industry. However, the majority of infections, especially community-acquired 13 , remain susceptible to cheap generic antibiotics even today, and the probability of a new blockbuster drug that would garner a large market share is progressively in decline 290 . This and multiple other factors, including increased costs for registration trials, a regulatory environment and challenges in clinical trial design, have led many pharmaceutical firms to abandon antibiotic development, as it offers a poor return on investment 291 .
Nevertheless, the paradigm for antibiotic development has shifted and, since the 2000s, niche antibiotics, particularly for gram-negative pathogens, have progressively emerged, developed by small biotech companies. These niche antibiotics specifically address gaps in standard antibiotic treatment coverage, yet leverage high prices to compensate for a small market share. The future success of these niche antibiotics could be increased by the emergence of rapid diagnostic tests that can detect specific pathogens or specific resistance markers immediately.
New antibiotics
The first generation of niche antibiotics were new β-lactams or BLIs developed for individual MDR or XDR pathogens 292 . The greatest unmet need for pneumonia due to gram-negative pathogens is for treatment of carbapenem-resistant Acinetobacter spp.; the only agent in development specifically for Acinetobacter spp. is a combination of two BLIs 293 . Both BLIs also have intrinsic β-lactam activity but are being studied in combination with a carbapenem for serious Acinetobacter spp. infections, including pneumonia.
Agents specific for Pseudomonas spp. are also in development. Murepavadin is the first of a new class of antibiotics that inhibit the outer membrane assembly of P. aeruginosa ; other drugs targeting outer membrane assembly are in development, including phage-derived endolysins 294 . Small molecule inhibitors of the type-III secretion apparatus in P. aeruginosa , a crucial component of its pathogenesis, are also in development.
One exception to the niche drug approach is cefiderocol, an extremely broad-spectrum agent with activity against almost all MDR pathogens. Cefiderocol links ceftazidime and cefepime together, maintaining the β-lactam bactericidal mechanism whilst enhancing bacterial uptake 295 . Bacteria take up cefiderocol through iron channels, and this mechanism is extremely appealing, as many MDR gram-negative pathogens, including P. aeruginosa , Acinetobacter spp. and Stenotrophomonas spp., avidly take up iron, and a major component of the acute-phase host response is to sequester iron from pathogens. Cefiderocol was non-inferior to high-dose extended-infusion meropenem for HAP due to gram-negative pathogens 296 , but it was associated with a higher mortality than the best available therapy for pneumonia and bacteraemia, specifically due to carbapenem-resistant Acinetobacter spp. 292 .
Lefamulin is the first truly new antibiotic class since the oxazolidinone linezolid. The mechanism of action of lefamulin is via protein synthesis inhibition, and lefamulin is approved for the treatment of CAP based on equivalence to moxifloxacin 297 , 298 . This drug can be used as a single agent to target MRSA and other CAP pathogens resistant to macrolide, β-lactam and fluoroquinolone antibiotics, and possibly in cases of treatment failure and/or in patients with multiple drug allergies. Unfortunately, lefamulin does not have substantial activity against ESBL-producing gram-negative pathogens, which is an unmet need in CAP.
Non-antibiotic therapy
Monoclonal or polyclonal antibodies to specific MDR pathogens, including S. aureus and P. aeruginosa , are the ultimate narrow-spectrum agents, being both extremely safe and having the great advantage of not disturbing the commensal microbiota 299 , 300 . Antibodies against the P. aeruginosa type-III secretion apparatus, alginate and other unique targets have entered clinical trials. Several anti- S. aureus antibodies have also been developed 301 . The challenge for specific antibodies is whether they should be used for prevention or as adjuncts to antibiotic therapy. The lack of sensitive risk factors or predictive markers for pneumonia caused by a specific pathogen make prophylactic trials difficult and potential clinical use expensive; thus, development for preventive indications has been abandoned for several agents, and attention has shifted to adjunctive use, despite this being associated with loss of the microbiota-sparing effect with this strategy.
Case reports have been published on bacteriophage therapy as an alternative to antibiotics in patients with extremely difficult-to-treat pneumonia 302 . However, major logistic issues must be overcome before phage therapy becomes a legitimate option 303 : the individual patient’s isolate must be tested for susceptibility against a battery of bacteria-specific phages; a cocktail of at least three phages is usually needed, owing to the emergence of resistance to any single phage; and the availability of phages and susceptibility testing facilities remain extremely limited. Furthermore, the optimal delivery method, namely aerosolization, instillation or venous infusion, remains unclear. No large-scale clinical trials have been completed.
Lastly, the COVID-19 pandemic has generated a large number of studies of adjuvant treatments focusing on host response to SARS-CoV-2. It remains unclear whether any adjuvant treatments other than corticosteroids that may provide benefit in SARS-CoV-2 infection can be used for influenza or other serious viral pneumonias. However, the COVID-19 pandemic has clearly increased interest in both host-directed therapy and newer antivirals.
Mandell, L. A. & Niederman, M. S. Aspiration pneumonia. N. Engl. J. Med. 380 , 651–663 (2019). A review article about aspiration pneumonia, including new insights about microbial aetiology and antibiotic treatment .
CAS PubMed Google Scholar
Cillóniz, C. et al. Microbial aetiology of community-acquired pneumonia and its relation to severity. Thorax 66 , 340–346 (2011).
PubMed Google Scholar
Torres, A. et al. International ERS/ESICM/ESCMID/ALAT guidelines for the management of hospital-acquired pneumonia and ventilator-associated pneumonia. Eur. Respir. J. 50 , 1700582 (2017). In these international European and Latin American guidelines, a panel of experts present recommendations about diagnosis, risk factor for antibiotic resistance and type and duration of treatment for HAP and VAP. PICO questions and GRADE methodology were used .
Kalil, A. C. et al. Management of adults with hospital-acquired and ventilator-associated pneumonia: 2016 clinical practice guidelines by the Infectious Diseases Society of America and the American Thoracic Society. Clin. Infect. Dis. 63 , e61–e111 (2016). These guidelines provide risk factors for suspected MDR or XDR microorganisms and recommendations for empirical treatments, use of biomarkers and duration of antibiotic administration .
PubMed PubMed Central Google Scholar
Metlay, J. P. et al. Diagnosis and treatment of adults with community-acquired pneumonia. An Official Clinical Practice Guideline of the American Thoracic Society and Infectious Diseases Society of America. Am. J. Respir. Crit. Care Med. 200 , e45–e67 (2019). These guidelines include new recommendations for microbiological diagnostic tests, in particular for empirical treatments in outside and in-hospital patients .
Prina, E., Ranzani, O. T. & Torres, A. Community-acquired pneumonia. Lancet 386 , 1097–1108 (2015).
Di Pasquale, M. F. et al. Prevalence and etiology of community-acquired pneumonia in immunocompromised patients. Clin. Infect. Dis. 68 , 1482–1493 (2019).
GBD 2019 Diseases and Injuries Collaborators. Global burden of 369 diseases and injuries in 204 countries and territories, 1990-2019: a systematic analysis for the Global Burden of Disease Study 2019. Lancet 396 , 1204–1222 (2020).
Google Scholar
GBD 2016 Lower Respiratory Infections Collaborators. Estimates of the global, regional, and national morbidity, mortality, and aetiologies of lower respiratory infections in 195 countries, 1990-2016: a systematic analysis for the Global Burden of Disease Study 2016. Lancet Infect. Dis. 18 , 1191–1210 (2018).
Komiya, K. et al. Prognostic implications of aspiration pneumonia in patients with community acquired pneumonia: a systematic review with meta-analysis. Sci. Rep. 6 , 38097 (2016).
CAS PubMed PubMed Central Google Scholar
Lindenauer, P. K. et al. Variation in the diagnosis of aspiration pneumonia and association with hospital pneumonia outcomes. Ann. Am. Thorac. Soc. 15 , 562–569 (2018).
Neill, S. & Dean, N. Aspiration pneumonia and pneumonitis: a spectrum of infectious/noninfectious diseases affecting the lung. Curr. Opin. Infect. Dis. 32 , 152–157 (2019).
Jain, S. et al. Community-acquired pneumonia requiring hospitalization among U.S. adults. N. Engl. J. Med. 373 , 415–427 (2015).
Torres, A., Peetermans, W. E., Viegi, G. & Blasi, F. Risk factors for community-acquired pneumonia in adults in Europe: a literature review. Thorax 68 , 1057–1065 (2013).
Norris, T., Vahratian, A. & Cohen, R. A. Vaccination coverage among adults aged 65 and over: United States, 2015. NCHS Data Brief No. 281 (CDC, 2017).
Fedson, D. S. et al. Pneumococcal polysaccharide vaccination for adults: new perspectives for Europe. Expert. Rev. Vaccines 10 , 1143–1167 (2011).
Jamal, A. et al. Current cigarette smoking among adults – United States, 2016. MMWR 67 , 53–59 (2018).
Louie, J. K. et al. Factors associated with death or hospitalization due to pandemic 2009 influenza A(H1N1) infection in California. JAMA 302 , 1896–1902 (2009).
Wu, C. et al. Risk factors associated with acute respiratory distress syndrome and death in patients with coronavirus disease 2019 pneumonia in Wuhan, China. JAMA Intern. Med. 180 , 934–943 (2020).
Barbier, F., Andremont, A., Wolff, M. & Bouadma, L. Hospital-acquired pneumonia and ventilator-associated pneumonia: recent advances in epidemiology and management. Curr. Opin. Pulm. Med. 19 , 216–228 (2013).
Rosenthal, V. D. et al. International Nosocomial Infection Control Consortium (INICC) report, data summary of 36 countries, for 2004-2009. Am. J. Infect. Control. 40 , 396–407 (2012).
Giuliano, K. K., Baker, D. & Quinn, B. The epidemiology of nonventilator hospital-acquired pneumonia in the United States. Am. J. Infect. Control. 46 , 322–327 (2018).
Bonell, A. et al. A systematic review and meta-analysis of ventilator-associated pneumonia in adults in Asia: an analysis of national income level on incidence and etiology. Clin. Infect. Dis. 68 , 511–518 (2019).
Bouadma, L. et al. Ventilator-associated events: prevalence, outcome, and relationship with ventilator-associated pneumonia. Crit. Care Med. 43 , 1798–1806 (2015).
Shi, T. et al. Global and regional burden of hospital admissions for pneumonia in older adults: a systematic review and meta-analysis. J. Infect. Dis. 222 , S570–S576 (2020).
GBD 2017 Causes of Death Collaborators. Global, regional, and national age-sex-specific mortality for 282 causes of death in 195 countries and territories, 1980-2017: a systematic analysis for the Global Burden of Disease Study 2017. Lancet 392 , 1736–1788 (2018).
JustActions. The Missing Piece. Why Continued Neglect of Pneumonia Threatens the Achivement of Health Goals (JustActions, 2018).
Nunes, B. P., Flores, T. R., Mielke, G. I., Thumé, E. & Facchini, L. A. Multimorbidity and mortality in older adults: a systematic review and meta-analysis. Arch. Gerontol. Geriatr. 67 , 130–138 (2016).
Arnold, F. W. et al. Mortality differences among hospitalized patients with community-acquired pneumonia in three world regions: results from the Community-Acquired Pneumonia Organization (CAPO) International Cohort Study. Respir. Med. 107 , 1101–1111 (2013).
Heo, J. Y. & Song, J. Y. Disease burden and etiologic distribution of community-acquired pneumonia in adults: evolving epidemiology in the era of pneumococcal conjugate vaccines. Infect. Chemother. 50 , 287–300 (2018).
Cillóniz, C. et al. Community-acquired pneumonia in outpatients: aetiology and outcomes. Eur. Respir. J. 40 , 931–938 (2012).
Luna, C. M. et al. The impact of age and comorbidities on the mortality of patients of different age groups admitted with community-acquired pneumonia. Ann. Am. Thorac. Soc. 13 , 1519–1526 (2016).
Cillóniz, C. et al. Twenty-year trend in mortality among hospitalized patients with pneumococcal community-acquired pneumonia. PLoS ONE 13 , e0200504 (2018).
Corrales-Medina, V. F. et al. Intermediate and long-term risk of new-onset heart failure after hospitalization for pneumonia in elderly adults. Am. Heart J. 170 , 306–312 (2015).
Eurich, D. T., Marrie, T. J., Minhas-Sandhu, J. K. & Majumdar, S. R. Ten-year mortality after community-acquired pneumonia. A prospective cohort. Am. J. Respir. Crit. Care Med. 192 , 597–604 (2015).
Ramirez, J. A. et al. Adults hospitalized with pneumonia in the United States: incidence, epidemiology and mortality. Clin. Infect. Dis. 65 , 1806–1812 (2017).
Bordon, J. et al. Hospitalization due to community-acquired pneumonia in patients with chronic obstructive pulmonary disease: incidence, epidemiology and outcomes. Clin. Microbiol. Infect. 26 , 220–226 (2020).
Torres, A. et al. Burden of pneumococcal community-acquired pneumonia in adults across Europe: a literature review. Respir. Med. 137 , 6–13 (2018).
Magill, S. S. et al. Multistate point-prevalence survey of health care-associated infections. N. Engl. J. Med. 370 , 1198–1208 (2014).
Micek, S. T., Chew, B., Hampton, N. & Kollef, M. H. A case-control study assessing the impact of nonventilated hospital-acquired pneumonia on patient outcomes. Chest 150 , 1008–1014 (2016).
Melsen, W. G. et al. Attributable mortality of ventilator-associated pneumonia: a meta-analysis of individual patient data from randomised prevention studies. Lancet Infect. Dis. 13 , 665–671 (2013).
Bassetti, M. et al. Risk stratification and treatment of ICU-acquired pneumonia caused by multidrug-resistant/extensively drug-resistant/pandrug-resistant bacteria. Curr. Opin. Crit. Care 24 , 385–393 (2018).
Herkel, T. et al. Epidemiology of hospital-acquired pneumonia: results of a Central European multicenter, prospective, observational study compared with data from the European region. Biomed. Pap. Med. Fac. Univ. Palacky. Olomouc Czech Repub. 160 , 448–455 (2016).
Ibn Saied, W. et al. A comparison of the mortality risk associated with ventilator-acquired bacterial pneumonia and nonventilator ICU-acquired bacterial pneumonia. Crit. Care Med. 47 , 345–352 (2019).
Talbot, G. H. et al. Evidence-based study design for hospital-acquired bacterial pneumonia and ventilator-associated bacterial pneumonia. J. Infect. Dis. 219 , 1536–1544 (2019).
McAllister, D. A. et al. Global, regional, and national estimates of pneumonia morbidity and mortality in children younger than 5 years between 2000 and 2015: a systematic analysis. Lancet Glob. Health 7 , e47–e57 (2019).
Weir, D. L., Majumdar, S. R., McAlister, F. A., Marrie, T. J. & Eurich, D. T. The impact of multimorbidity on short-term events in patients with community-acquired pneumonia: prospective cohort study. Clin. Microbiol. Infect. 21 , 264.e7–264.e13 (2015).
CAS Google Scholar
Bradley, J. S. et al. The management of community-acquired pneumonia in infants and children older than 3 months of age: clinical practice guidelines by the Pediatric Infectious Diseases Society and the Infectious Diseases Society of America. Clin. Infect. Dis. 53 , e25–e76 (2011).
Barbagelata, E. et al. Gender differences in community-acquired pneumonia. Minerva Med. 111 , 153–165 (2020).
Mutepe, N. D. et al. Effects of cigarette smoke condensate on pneumococcal biofilm formation and pneumolysin. Eur. Respir. J. 41 , 392–395 (2013).
Samokhvalov, A. V., Irving, H. M. & Rehm, J. Alcohol consumption as a risk factor for pneumonia: a systematic review and meta-analysis. Epidemiol. Infect. 138 , 1789–1795 (2010).
Neupane, B. et al. Long-term exposure to ambient air pollution and risk of hospitalization with community-acquired pneumonia in older adults. Am. J. Respir. Crit. Care Med. 181 , 47–53 (2010).
American Thoracic Society & Infectious Diseases Society of America. Guidelines for the management of adults with hospital-acquired, ventilator-associated, and healthcare-associated pneumonia. Am. J. Respir. Crit. Care Med. 171 , 388–416 (2005).
Le, M. N.-T. et al. Oral colonisation by antimicrobial-resistant Gram-negative bacteria among long-term care facility residents: prevalence, risk factors, and molecular epidemiology. Antimicrob. Resist. Infect. Control. 9 , 45 (2020).
Feldman, C. et al. The presence and sequence of endotracheal tube colonization in patients undergoing mechanical ventilation. Eur. Respir. J. 13 , 546–551 (1999).
Cilloniz, C. et al. Seasonality of pathogens causing community-acquired pneumonia. Respirology 22 , 778–785 (2017).
Para, R. A., Fomda, B. A., Jan, R. A., Shah, S. & Koul, P. A. Microbial etiology in hospitalized North Indian adults with community-acquired pneumonia. Lung India 35 , 108–115 (2018).
Tao, L.-L. et al. Etiology and antimicrobial resistance of community-acquired pneumonia in adult patients in China. Chin. Med. J. 125 , 2967–2972 (2012).
Shoar, S. & Musher, D. M. Etiology of community-acquired pneumonia in adults: a systematic review. Pneumonia 12 , 11 (2020).
Moberley, S., Holden, J., Tatham, D. P. & Andrews, R. M. Vaccines for preventing pneumococcal infection in adults. Cochrane Database Syst. Rev. 2013 , CD000422 (2013).
PubMed Central Google Scholar
Centers for Disease Control and Prevention (CDC). Current cigarette smoking among adults - United States, 2011. MMWR 61 , 889–894 (2012).
Luca, D. L. et al. Impact of pneumococcal vaccination on pneumonia hospitalizations and related costs in Ontario: a population-based ecological study. Clin. Infect. Dis. 66 , 541–547 (2017).
Johansson, N., Kalin, M., Tiveljung-Lindell, A., Giske, C. G. & Hedlund, J. Etiology of community-acquired pneumonia: increased microbiological yield with new diagnostic methods. Clin. Infect. Dis. 50 , 202–209 (2010).
Rozenbaum, M. H. et al. The role of Streptococcus pneumoniae in community-acquired pneumonia among adults in Europe: a meta-analysis. Eur. J. Clin. Microbiol. Infect. Dis. 32 , 305–316 (2013).
Huijts, S. M. et al. Diagnostic accuracy of a serotype-specific antigen test in community-acquired pneumonia. Eur. Respir. J. 42 , 1283–1290 (2013).
Aliberti, S. et al. Multidrug-resistant pathogens in hospitalised patients coming from the community with pneumonia: a European perspective. Thorax 68 , 997–999 (2013).
Shindo, Y. et al. Risk factors for drug-resistant pathogens in community-acquired and healthcare-associated pneumonia. Am. J. Respir. Crit. Care Med. 188 , 985–995 (2013).
Prina, E. et al. Risk factors associated with potentially antibiotic-resistant pathogens in community-acquired pneumonia. Ann. Am. Thorac. Soc. 12 , 153–160 (2015).
Ceccato, A. et al. Validation of a prediction score for drug-resistant microorganisms in community-acquired pneumonia. Ann. Am. Thorac. Soc. 18 , 257–265 (2021).
Cilloniz, C. et al. Difficult to treat microorganisms in patients over 80 years with community-acquired pneumonia: the prevalence of PES pathogens. Eur. Respir. J. 56 , 2000773 (2020).
Webb, B. J. et al. Derivation and multicenter validation of the drug resistance in pneumonia clinical prediction score. Antimicrob. Agents Chemother. 60 , 2652–2663 (2016).
Karhu, J., Ala-Kokko, T. I., Vuorinen, T., Ohtonen, P. & Syrjälä, H. Lower respiratory tract virus findings in mechanically ventilated patients with severe community-acquired pneumonia. Clin. Infect. Dis. 59 , 62–70 (2014).
Wu, X. et al. Incidence of respiratory viral infections detected by PCR and real-time PCR in adult patients with community-acquired pneumonia: a meta-analysis. Respiration 89 , 343–352 (2015).
Zhou, F. et al. Disease severity and clinical outcomes of community acquired pneumonia caused by non-influenza respiratory viruses in adults: a multicenter prospective registry study from CAP-China Network. Eur. Respir. J. 54 , 1802406 (2019).
Cillóniz, C. et al. Pure viral sepsis secondary to community-acquired pneumonia in adults: risk and prognostic factors. J. Infect. Dis. 220 , 1166–1171 (2019).
Jain, S. et al. Community-acquired pneumonia requiring hospitalization among U.S. children. N. Engl. J. Med. 372 , 835–845 (2015). This study is a prospective multicentre investigation of the CAP microbial aetiology in hospitalized patients. Very importantly, PCR tests for the detection of viral pathogens , Legionella spp. and Mycoplasma pneumoniae were systematically used in the diagnostic work-up. With this approach, viruses represented the first cause of CAP .
Weber, D. J. et al. Microbiology of ventilator-associated pneumonia compared with that of hospital-acquired pneumonia. Infect. Control. Hosp. Epidemiol. 28 , 825–831 (2007).
Magiorakos, A.-P. et al. Multidrug-resistant, extensively drug-resistant and pandrug-resistant bacteria: an international expert proposal for interim standard definitions for acquired resistance. Clin. Microbiol. Infect. 18 , 268–281 (2012).
Parker, D., Ahn, D., Cohen, T. & Prince, A. Innate immune signaling activated by MDR bacteria in the airway. Physiol. Rev. 96 , 19–53 (2016).
Grousd, J. A., Rich, H. E. & Alcorn, J. F. Host-pathogen interactions in gram-positive bacterial pneumonia. Clin. Microbiol. Rev. 32 , e00107-18 (2019).
Kutter, J. S., Spronken, M. I., Fraaij, P. L., Fouchier, R. A. & Herfst, S. Transmission routes of respiratory viruses among humans. Curr. Opin. Virol. 28 , 142–151 (2018).
Siegel, S. J. & Weiser, J. N. Mechanisms of bacterial colonization of the respiratory tract. Annu. Rev. Microbiol. 69 , 425–444 (2015).
Quinton, L. J., Walkey, A. J. & Mizgerd, J. P. Integrative physiology of pneumonia. Physiol. Rev. 98 , 1417–1464 (2018).
Dickson, R. P., Erb-Downward, J. R. & Huffnagle, G. B. Towards an ecology of the lung: new conceptual models of pulmonary microbiology and pneumonia pathogenesis. Lancet Respir. Med. 2 , 238–246 (2014). A review–opinion article about new insights into the aetiopathogenesis of pneumonia based on changes in the microbiota .
Pettigrew, M. M., Tanner, W. & Harris, A. D. The lung microbiome and pneumonia. J. Infect. Dis. https://doi.org/10.1093/infdis/jiaa702 (2020).
Article Google Scholar
Brown, R. L., Sequeira, R. P. & Clarke, T. B. The microbiota protects against respiratory infection via GM-CSF signaling. Nat. Commun. 8 , 1512 (2017).
Nishimoto, A. T., Rosch, J. W. & Tuomanen, E. I. Pneumolysin: pathogenesis and therapeutic target. Front. Microbiol. 11 , 1543 (2020).
von Hoven, G., Qin, Q., Neukirch, C., Husmann, M. & Hellmann, N. Staphylococcus aureus α-toxin: small pore, large consequences. Biol. Chem. 400 , 1261–1276 (2019).
Hauser, A. R. The type III secretion system of Pseudomonas aeruginosa: infection by injection. Nat. Rev. Microbiol. 7 , 654–665 (2009).
Ferguson, N. D. et al. The Berlin definition of ARDS: an expanded rationale, justification, and supplementary material. Intensive Care Med. 38 , 1573–1582 (2012).
Matthay, M. A. et al. Acute respiratory distress syndrome. Nat. Rev. Dis. Prim. 5 , 18 (2019).
Whitsett, J. A. & Alenghat, T. Respiratory epithelial cells orchestrate pulmonary innate immunity. Nat. Immunol. 16 , 27–35 (2015).
Cheng, D. et al. Airway epithelium controls lung inflammation and injury through the NF-κB pathway. J. Immunol. 178 , 6504–6513 (2007).
Quinton, L. J. et al. Functions and regulation of NF-κB RelA during pneumococcal pneumonia. J. Immunol. 178 , 1896–1903 (2007).
Han, S. & Mallampalli, R. K. The role of surfactant in lung disease and host defense against pulmonary infections. Ann. Am. Thorac. Soc. 12 , 765–774 (2015).
Carey, R. M. & Lee, R. J. Taste receptors in upper airway innate immunity. Nutrients 11 , 2017 (2019).
CAS PubMed Central Google Scholar
Lee, R. J. & Cohen, N. A. The emerging role of the bitter taste receptor T2R38 in upper respiratory infection and chronic rhinosinusitis. Am. J. Rhinol. Allergy 27 , 283–286 (2013).
McAleer, J. P. & Kolls, J. K. Directing traffic: IL-17 and IL-22 coordinate pulmonary immune defense. Immunol. Rev. 260 , 129–144 (2014).
Aujla, S. J. et al. IL-22 mediates mucosal host defense against Gram-negative bacterial pneumonia. Nat. Med. 14 , 275–281 (2008).
Allard, B., Panariti, A. & Martin, J. G. Alveolar macrophages in the resolution of inflammation, tissue repair, and tolerance to infection. Front. Immunol. 9 , 1777 (2018).
Preston, J. A. et al. Alveolar macrophage apoptosis-associated bacterial killing helps prevent murine pneumonia. Am. J. Respir. Crit. Care Med. 200 , 84–97 (2019).
González-Juarbe, N. et al. Pore-forming toxins induce macrophage necroptosis during acute bacterial pneumonia. PLoS Pathog. 11 , e1005337 (2015).
Kitur, K. et al. Toxin-induced necroptosis is a major mechanism of Staphylococcus aureus lung damage. PLoS Pathog. 11 , e1004820 (2015).
Kitur, K. et al. Necroptosis promotes Staphylococcus aureus clearance by inhibiting excessive inflammatory signaling. Cell Rep. 16 , 2219–2230 (2016).
Panda, S. K. & Colonna, M. Innate lymphoid cells in mucosal immunity. Front. Immunol. 10 , 861 (2019).
Kaiko, G. E., Phipps, S., Angkasekwinai, P., Dong, C. & Foster, P. S. NK cell deficiency predisposes to viral-induced Th2-type allergic inflammation via epithelial-derived IL-25. J. Immunol. 185 , 4681–4690 (2010).
Jayaraman, A. et al. IL-15 complexes induce NK- and T-cell responses independent of type I IFN signaling during rhinovirus infection. Mucosal Immunol. 7 , 1151–1164 (2014).
Van Maele, L. et al. Activation of type 3 innate lymphoid cells and interleukin 22 secretion in the lungs during Streptococcus pneumoniae infection. J. Infect. Dis. 210 , 493–503 (2014).
Xiong, H. et al. Innate lymphocyte/ly6c(hi) monocyte crosstalk promotes Klebsiella pneumoniae clearance. Cell 165 , 679–689 (2016).
Hinks, T. S. C. et al. Steroid-induced deficiency of mucosal-associated invariant T cells in the chronic obstructive pulmonary disease lung. Implications for nontypeable Haemophilus influenzae infection. Am. J. Respir. Crit. Care Med. 194 , 1208–1218 (2016).
Meierovics, A. I. & Cowley, S. C. MAIT cells promote inflammatory monocyte differentiation into dendritic cells during pulmonary intracellular infection. J. Exp. Med. 213 , 2793–2809 (2016).
Liu, J. et al. Advanced role of neutrophils in common respiratory diseases. J. Immunol. Res. 2017 , 6710278 (2017).
Castanheira, F. V. S. & Kubes, P. Neutrophils and NETs in modulating acute and chronic inflammation. Blood 133 , 2178–2185 (2019).
Xiong, H. et al. Distinct contributions of neutrophils and CCR2+ monocytes to pulmonary clearance of different Klebsiella pneumoniae strains. Infect. Immun. 83 , 3418–3427 (2015).
Winter, C. et al. Important role for CC chemokine ligand 2-dependent lung mononuclear phagocyte recruitment to inhibit sepsis in mice infected with Streptococcus pneumoniae. J. Immunol. 182 , 4931–4937 (2009).
Weber, G. F. et al. Pleural innate response activator B cells protect against pneumonia via a GM-CSF-IgM axis. J. Exp. Med. 211 , 1243–1256 (2014).
de Stoppelaar, S. F. et al. Thrombocytopenia impairs host defense in gram-negative pneumonia-derived sepsis in mice. Blood 124 , 3781–3790 (2014).
van den Boogaard, F. E. et al. Thrombocytopenia impairs host defense during murine Streptococcus pneumoniae pneumonia. Crit. Care Med. 43 , e75–e83 (2015).
Ichinohe, T. et al. Microbiota regulates immune defense against respiratory tract influenza A virus infection. Proc. Natl Acad. Sci. USA 108 , 5354–5359 (2011).
Schuijt, T. J. et al. The gut microbiota plays a protective role in the host defence against pneumococcal pneumonia. Gut 65 , 575–583 (2016).
Haak, B. W. & Wiersinga, W. J. The role of the gut microbiota in sepsis. Lancet Gastroenterol. Hepatol. 2 , 135–143 (2017).
Netea, M. G., Schlitzer, A., Placek, K., Joosten, L. A. B. & Schultze, J. L. Innate and adaptive immune memory: an evolutionary continuum in the host’s response to pathogens. Cell Host Microbe 25 , 13–26 (2019).
Giamarellos-Bourboulis, E. J. et al. Activate: randomized clinical trial of BCG vaccination against infection in the elderly. Cell 183 , 315–323.e9 (2020).
Hwang, J. Y., Randall, T. D. & Silva-Sanchez, A. Inducible bronchus-associated lymphoid tissue: taming inflammation in the lung. Front. Immunol. 7 , 258 (2016).
Snyder, M. E. & Farber, D. L. Human lung tissue resident memory T cells in health and disease. Curr. Opin. Immunol. 59 , 101–108 (2019).
Smith, N. M. et al. Regionally compartmentalized resident memory T cells mediate naturally acquired protection against pneumococcal pneumonia. Mucosal Immunol. 11 , 220–235 (2018).
Serhan, C. N. & Levy, B. D. Resolvins in inflammation: emergence of the pro-resolving superfamily of mediators. J. Clin. Invest. 128 , 2657–2669 (2018).
Flitter, B. A. et al. Pseudomonas aeruginosa sabotages the generation of host proresolving lipid mediators. Proc. Natl Acad. Sci. USA 114 , 136–141 (2017).
Sham, H. P. et al. 15-epi-lipoxin A4, resolvin D2, and resolvin D3 induce NF-κB regulators in bacterial pneumonia. J. Immunol. 200 , 2757–2766 (2018).
Zemans, R. L. et al. Neutrophil transmigration triggers repair of the lung epithelium via β-catenin signaling. Proc. Natl Acad. Sci. USA 108 , 15990–15995 (2011).
Liu, Y. et al. FoxM1 mediates the progenitor function of type II epithelial cells in repairing alveolar injury induced by Pseudomonas aeruginosa. J. Exp. Med. 208 , 1473–1484 (2011).
Kumar, P. A. et al. Distal airway stem cells yield alveoli in vitro and during lung regeneration following H1N1 influenza infection. Cell 147 , 525–538 (2011).
Matsuzaki, Y. et al. Stat3 is required for cytoprotection of the respiratory epithelium during adenoviral infection. J. Immunol. 177 , 527–537 (2006).
Quinton, L. J. et al. Alveolar epithelial STAT3, IL-6 family cytokines, and host defense during Escherichia coli pneumonia. Am. J. Respir. Cell Mol. Biol. 38 , 699–706 (2008).
Quinton, L. J. et al. Leukemia inhibitory factor signaling is required for lung protection during pneumonia. J. Immunol. 188 , 6300–6308 (2012).
Poe, S. L. et al. STAT1-regulated lung MDSC-like cells produce IL-10 and efferocytose apoptotic neutrophils with relevance in resolution of bacterial pneumonia. Mucosal Immunol. 6 , 189–199 (2013).
D’Alessio, F. R. et al. CD4+CD25+Foxp3+ Tregs resolve experimental lung injury in mice and are present in humans with acute lung injury. J. Clin. Invest. 119 , 2898–2913 (2009).
Monticelli, L. A. et al. Innate lymphoid cells promote lung-tissue homeostasis after infection with influenza virus. Nat. Immunol. 12 , 1045–1054 (2011).
Laidlaw, B. J. et al. CD4+ T cell help guides formation of CD103+ lung-resident memory CD8+ T cells during influenza viral infection. Immunity 41 , 633–645 (2014).
Xu, X. et al. Conventional NK cells can produce IL-22 and promote host defense in Klebsiella pneumoniae pneumonia. J. Immunol. 192 , 1778–1786 (2014).
Kradin, R. L. & Digumarthy, S. The pathology of pulmonary bacterial infection. Semin. Diagn. Pathol. 34 , 498–509 (2017).
Pritt, B. S. & Aubry, M. C. Histopathology of viral infections of the lung. Semin. Diagn. Pathol. 34 , 510–517 (2017).
Singer, M. et al. The third international consensus definitions for sepsis and septic shock (Sepsis-3). JAMA 315 , 801–810 (2016).
Angus, D. C. & van der Poll, T. Severe sepsis and septic shock. N. Engl. J. Med. 369 , 840–851 (2013).
Dremsizov, T. et al. Severe sepsis in community-acquired pneumonia: when does it happen, and do systemic inflammatory response syndrome criteria help predict course? Chest 129 , 968–978 (2006).
Giuliano, K. K. & Baker, D. Sepsis in the context of nonventilator hospital-acquired pneumonia. Am. J. Crit. Care 29 , 9–14 (2020).
Hotchkiss, R. S. et al. Sepsis and septic shock. Nat. Rev. Dis. Prim. 2 , 16045 (2016).
van der Poll, T., van de Veerdonk, F. L., Scicluna, B. P. & Netea, M. G. The immunopathology of sepsis and potential therapeutic targets. Nat. Rev. Immunol. 17 , 407–420 (2017).
Corrales-Medina, V. F., Musher, D. M., Shachkina, S. & Chirinos, J. A. Acute pneumonia and the cardiovascular system. Lancet 381 , 496–505 (2013).
Corrales-Medina, V. F. et al. Association between hospitalization for pneumonia and subsequent risk of cardiovascular disease. JAMA 313 , 264–274 (2015). The short-term and long-term risk of cardiovascular diseases after CAP hospitalization is shown in this capital study .
Corrales-Medina, V. F. et al. Cardiac complications in patients with community-acquired pneumonia: a systematic review and meta-analysis of observational studies. PLoS Med. 8 , e1001048 (2011).
Violi, F. et al. Cardiovascular complications and short-term mortality risk in community-acquired pneumonia. Clin. Infect. Dis. 64 , 1486–1493 (2017).
Ramirez, J. et al. Acute myocardial infarction in hospitalized patients with community-acquired pneumonia. Clin. Infect. Dis. 47 , 182–187 (2008).
Mortensen, E. M. et al. Causes of death for patients with community-acquired pneumonia: results from the Pneumonia Patient Outcomes Research Team cohort study. Arch. Intern. Med. 162 , 1059–1064 (2002).
Musher, D. M., Abers, M. S. & Corrales-Medina, V. F. Acute infection and myocardial infarction. N. Engl. J. Med. 380 , 171–176 (2019). A review article showing the evidence of acute respiratory viral infection and the increased risk of myocardial infarction .
Milbrandt, E. B. et al. Prevalence and significance of coagulation abnormalities in community-acquired pneumonia. Mol. Med. 15 , 438–445 (2009).
van Vught, L. A. et al. Comparative analysis of the host response to community-acquired and hospital-acquired pneumonia in critically Ill patients. Am. J. Respir. Crit. Care Med. 194 , 1366–1374 (2016).
Naghavi, M. et al. Influenza infection exerts prominent inflammatory and thrombotic effects on the atherosclerotic plaques of apolipoprotein E-deficient mice. Circulation 107 , 762–768 (2003).
Madjid, M., Vela, D., Khalili-Tabrizi, H., Casscells, S. W. & Litovsky, S. Systemic infections cause exaggerated local inflammation in atherosclerotic coronary arteries: clues to the triggering effect of acute infections on acute coronary syndromes. Tex. Heart Inst. J. 34 , 11–18 (2007).
Jaw, J. E. et al. Lung exposure to lipopolysaccharide causes atherosclerotic plaque destabilisation. Eur. Respir. J. 48 , 205–215 (2016).
Yende, S. et al. Inflammatory markers at hospital discharge predict subsequent mortality after pneumonia and sepsis. Am. J. Respir. Crit. Care Med. 177 , 1242–1247 (2008).
Yende, S. et al. Elevated hemostasis markers after pneumonia increases one-year risk of all-cause and cardiovascular deaths. PLoS ONE 6 , e22847 (2011).
Iwashyna, T. J., Ely, E. W., Smith, D. M. & Langa, K. M. Long-term cognitive impairment and functional disability among survivors of severe sepsis. JAMA 304 , 1787–1794 (2010).
Shah, F. A. et al. Bidirectional relationship between cognitive function and pneumonia. Am. J. Respir. Crit. Care Med. 188 , 586–592 (2013).
Girard, T. D., Dittus, R. S. & Ely, E. W. Critical illness brain injury. Annu. Rev. Med. 67 , 497–513 (2016).
Chung, H.-Y., Wickel, J., Brunkhorst, F. M. & Geis, C. Sepsis-associated encephalopathy: from delirium to dementia? J. Clin. Med. 9 , 703 (2020).
Prescott, H. C., Sjoding, M. W. & Iwashyna, T. J. Diagnoses of early and late readmissions after hospitalization for pneumonia. A systematic review. Ann. Am. Thorac. Soc. 11 , 1091–1100 (2014).
Dang, T. T., Majumdar, S. R., Marrie, T. J. & Eurich, D. T. Recurrent pneumonia: a review with focus on clinical epidemiology and modifiable risk factors in elderly patients. Drugs Aging 32 , 13–19 (2015).
Ekdahl, K., Braconier, J. H. & Svanborg, C. Immunoglobulin deficiencies and impaired immune response to polysaccharide antigens in adult patients with recurrent community-acquired pneumonia. Scand. J. Infect. Dis. 29 , 401–407 (1997).
Roquilly, A. et al. Alveolar macrophages are epigenetically altered after inflammation, leading to long-term lung immunoparalysis. Nat. Immunol. 21 , 636–648 (2020).
Lamping, D. L. et al. The community-acquired pneumonia symptom questionnaire: a new, patient-based outcome measure to evaluate symptoms in patients with community-acquired pneumonia. Chest 122 , 920–929 (2002).
Metlay, J. P. et al. Measuring symptomatic and functional recovery in patients with community-acquired pneumonia. J. Gen. Intern. Med. 12 , 423–430 (1997).
Chalmers, J. D. et al. Severity assessment tools for predicting mortality in hospitalised patients with community-acquired pneumonia. Systematic review and meta-analysis. Thorax 65 , 878–883 (2010).
Chalmers, J. D. et al. Risk factors for complicated parapneumonic effusion and empyema on presentation to hospital with community-acquired pneumonia. Thorax 64 , 556–558 (2009).
Falguera, M. et al. Predictive factors, microbiology and outcome of patients with parapneumonic effusion. Eur. Respir. J. 38 , 1173–1179 (2011).
Bhuiyan, M. U. et al. Combination of clinical symptoms and blood biomarkers can improve discrimination between bacterial or viral community-acquired pneumonia in children. BMC Pulmonary Med. 19 , 71 (2019).
Lhommet, C. et al. Predicting the microbial cause of community-acquired pneumonia: can physicians or a data-driven method differentiate viral from bacterial pneumonia at patient presentation? BMC Pulmonary Med. 20 , 62 (2020).
Torres, A., & Cillóniz, C. Clinical Management of Bacterial Pneumonia (Springer, 2015).
Cilloniz, C., Ceccato, A., San Jose, A. & Torres, A. Clinical management of community acquired pneumonia in the elderly patient. Expert Rev. Respir. Med. 10 , 1211–1220 (2016).
Schurink, C. A. M. et al. Clinical pulmonary infection score for ventilator-associated pneumonia: accuracy and inter-observer variability. Intensive Care Med. 30 , 217–224 (2004).
Fàbregas, N. et al. Clinical diagnosis of ventilator associated pneumonia revisited: comparative validation using immediate post-mortem lung biopsies. Thorax 54 , 867–873 (1999). The most complete immediate post-mortem study of VAP to validate clinical diagnosis .
Self, W. H., Courtney, D. M., McNaughton, C. D., Wunderink, R. G. & Kline, J. A. High discordance of chest x-ray and computed tomography for detection of pulmonary opacities in ED patients: implications for diagnosing pneumonia. Am. J. Emerg. Med. 31 , 401–405 (2013).
Laursen, C. B. et al. Diagnostic performance of chest X-ray for the diagnosis of community acquired pneumonia in acute admitted patients with respiratory symptoms. Scand. J. Trauma. Resusc. Emerg. Med. 21 , A21 (2013).
Claessens, Y.-E. et al. Early chest computed tomography scan to assist diagnosis and guide treatment decision for suspected community-acquired pneumonia. Am. J. Respir. Crit. Care Med. 192 , 974–982 (2015).
Ding, X., Xu, J., Zhou, J., Long, Q. & Chest, C. T. findings of COVID-19 pneumonia by duration of symptoms. Eur. J. Radiol. 127 , 109009 (2020).
Franquet, T. Imaging of community-acquired pneumonia. J. Thorac. Imaging 33 , 282–294 (2018).
D’Amato, M. et al. Assessment of thoracic ultrasound in complementary diagnosis and in follow up of community-acquired pneumonia (CAP). BMC Med. Imaging 17 , 52 (2017).
Long, L., Zhao, H.-T., Zhang, Z.-Y., Wang, G.-Y. & Zhao, H.-L. Lung ultrasound for the diagnosis of pneumonia in adults: a meta-analysis. Medicine 96 , e5713 (2017).
Mongodi, S. et al. Lung ultrasound for early diagnosis of ventilator-associated pneumonia. Chest 149 , 969–980 (2016).
Bouhemad, B., Dransart-Rayé, O., Mojoli, F. & Mongodi, S. Lung ultrasound for diagnosis and monitoring of ventilator-associated pneumonia. Ann. Transl. Med. 6 , 418 (2018).
Musher, D. M., Montoya, R. & Wanahita, A. Diagnostic value of microscopic examination of Gram-stained sputum and sputum cultures in patients with bacteremic pneumococcal pneumonia. Clin. Infect. Dis. 39 , 165–169 (2004).
Fukuyama, H., Yamashiro, S., Kinjo, K., Tamaki, H. & Kishaba, T. Validation of sputum Gram stain for treatment of community-acquired pneumonia and healthcare-associated pneumonia: a prospective observational study. BMC Infect. Dis. 14 , 534 (2014).
Ranzani, O. T. et al. Diagnostic accuracy of Gram staining when predicting staphylococcal hospital-acquired pneumonia and ventilator-associated pneumonia: a systematic review and meta-analysis. Clin. Microbiol. Infect. 26 , 1456–1463 (2020).
Torres, A., Artigas, A. & Ferrer, R. Biomarkers in the ICU: less is more? No. Intensive Care Med. 47 , 97–100 (2021).
Torres, A., Lee, N., Cilloniz, C., Vila, J. & Van der Eerden, M. Laboratory diagnosis of pneumonia in the molecular age. Eur. Respir. J. 48 , 1764–1778 (2016). In-depth revision of available molecular diagnostic techniques for bacterial and viral pneumonia .
Schulte, B. et al. Detection of pneumonia associated pathogens using a prototype multiplexed pneumonia test in hospitalized patients with severe pneumonia. PLoS ONE 9 , e110566 (2014).
Paonessa, J. R. et al. Rapid detection of methicillin-resistant Staphylococcus aureus in BAL: a pilot randomized controlled trial. Chest 155 , 999–1007 (2019).
Gastli, N. et al. Multicentric evaluation of BioFire FilmArray Pneumonia Panel for rapid bacteriological documentation of pneumonia. Clin. Microbiol. Infect. https://doi.org/10.1016/j.cmi.2020.11.014 (2020).
Article PubMed Google Scholar
Centers for Disease Control and Prevention. Overview of Testing for SARS-CoV-2 (COVID-19). https://www.cdc.gov/coronavirus/2019-ncov/hcp/testing-overview.html (CDC, 2020).
Karakioulaki, M. & Stolz, D. The case of procalcitonin for lower respiratory tract infections. BRN Rev. 5 , 277–293 (2019).
Krüger, S. et al. Inflammatory parameters predict etiologic patterns but do not allow for individual prediction of etiology in patients with CAP: results from the German Competence Network CAPNETZ. Respir. Res. 10 , 65 (2009).
Ramirez, P. et al. Sequential measurements of procalcitonin levels in diagnosing ventilator-associated pneumonia. Eur. Respir. J. 31 , 356–362 (2008).
Luyt, C.-E. et al. Usefulness of procalcitonin for the diagnosis of ventilator-associated pneumonia. Intensive Care Med. 34 , 1434–1440 (2008).
Schuetz, P. et al. Procalcitonin (PCT)-guided antibiotic stewardship: an international experts consensus on optimized clinical use. Clin. Chem. Lab. Med. 57 , 1308–1318 (2019).
Liapikou, A., Cilloniz, C. & Torres, A. Drugs that increase the risk of community-acquired pneumonia: a narrative review. Expert Opin. Drug Saf. 17 , 991–1003 (2018).
Niederman, M. S. et al. Efficacy and effectiveness of a 23-valent polysaccharide vaccine against invasive and non-invasive pneumococcal disease and related outcomes: a review of available evidence. Expert Rev Vaccines https://doi.org/10.1080/14760584.2021.1880328 (2021).
Maruyama, T. et al. Efficacy of 23-valent pneumococcal vaccine in preventing pneumonia and improving survival in nursing home residents: double blind, randomised and placebo controlled trial. BMJ 340 , c1004 (2010).
Falkenhorst, G. et al. Effectiveness of the 23-valent pneumococcal polysaccharide vaccine (PPV23) against pneumococcal disease in the elderly: systematic review and meta-analysis. PLoS ONE 12 , e0169368 (2017).
Bonten, M. J. M. et al. Polysaccharide conjugate vaccine against pneumococcal pneumonia in adults. N. Engl. J. Med. 372 , 1114–1125 (2015).
Patterson, S. et al. A post hoc assessment of duration of protection in CAPiTA (Community Acquired Pneumonia immunization Trial in Adults). Trials Vaccinol. 5 , 92–96 (2016).
Millar, E. V. et al. Indirect effect of 7-valent pneumococcal conjugate vaccine on pneumococcal colonization among unvaccinated household members. Clin. Infect. Dis. 47 , 989–996 (2008).
Hammitt, L. L. et al. Indirect effect of conjugate vaccine on adult carriage of Streptococcus pneumoniae: an explanation of trends in invasive pneumococcal disease. J. Infect. Dis. 193 , 1487–1494 (2006).
Chung, J. R. et al. Effects of influenza vaccination in the United States during the 2018-2019 influenza season. Clin. Infect. Dis. 71 , e368–e376 (2020).
Restivo, V. et al. Influenza vaccine effectiveness among high-risk groups: a systematic literature review and meta-analysis of case-control and cohort studies. Hum. Vaccin. Immunother. 14 , 724–735 (2018).
Chow, E. J. et al. Vaccine effectiveness against influenza-associated lower respiratory tract infections in hospitalized adults, Louisville, Kentucky, 2010-2013. Open. Forum Infect. Dis. 7 , ofaa262 (2020).
Lyons, P. G. & Kollef, M. H. Prevention of hospital-acquired pneumonia. Curr. Opin. Crit. Care 24 , 370–378 (2018).
Álvarez-Lerma, F. et al. Prevention of ventilator-associated pneumonia: the multimodal approach of the Spanish ICU “Pneumonia Zero” Program. Crit. Care Med. 46 , 181–188 (2018).
Palomar, M. et al. Impact of a national multimodal intervention to prevent catheter-related bloodstream infection in the ICU: the Spanish experience. Crit. Care Med. 41 , 2364–2372 (2013).
Ma, S. et al. A meta analysis of the effect of enhanced hand hygiene on the morbidity of ventilator-associated pneumonia. Zhonghua Wei Zhong Bing. Ji Jiu Yi Xue 26 , 304–308 (2014).
Drakulovic, M. B. et al. Supine body position as a risk factor for nosocomial pneumonia in mechanically ventilated patients: a randomised trial. Lancet 354 , 1851–1858 (1999).
Wang, L. et al. Semi-recumbent position versus supine position for the prevention of ventilator-associated pneumonia in adults requiring mechanical ventilation. Cochrane Database Syst. Rev. 2016 , CD009946 (2016).
Li Bassi, G. et al. Randomized, multicenter trial of lateral Trendelenburg versus semirecumbent body position for the prevention of ventilator-associated pneumonia. Intensive Care Med. 43 , 1572–1584 (2017).
Guérin, C. et al. Prone positioning in severe acute respiratory distress syndrome. N. Engl. J. Med. 368 , 2159–2168 (2013).
Douglas, I. S. et al. Safety and outcomes of prolonged usual care prone position mechanical ventilation to treat acute coronavirus disease 2019 hypoxemic respiratory failure. Crit. Care Med. 49 , 490–502 (2021).
Shelhamer, M. C. et al. Prone positioning in moderate to severe acute respiratory distress syndrome due to COVID-19: a cohort study and analysis of physiology. J. Intensive Care Med. 36 , 241–252 (2021).
Sud, S., Sud, M., Friedrich, J. O. & Adhikari, N. K. J. Effect of mechanical ventilation in the prone position on clinical outcomes in patients with acute hypoxemic respiratory failure: a systematic review and meta-analysis. CMAJ 178 , 1153–1161 (2008).
Mao, Z. et al. Subglottic secretion suction for preventing ventilator-associated pneumonia: an updated meta-analysis and trial sequential analysis. Crit. Care 20 , 353 (2016).
Marjanovic, N. et al. Multicentre randomised controlled trial to investigate the usefulness of continuous pneumatic regulation of tracheal cuff pressure for reducing ventilator-associated pneumonia in mechanically ventilated severe trauma patients: the AGATE study protocol. BMJ Open 7 , e017003 (2017).
Philippart, F. et al. Randomized intubation with polyurethane or conical cuffs to prevent pneumonia in ventilated patients. Am. J. Respir. Crit. Care Med. 191 , 637–645 (2015).
Klompas, M., Speck, K., Howell, M. D., Greene, L. R. & Berenholtz, S. M. Reappraisal of routine oral care with chlorhexidine gluconate for patients receiving mechanical ventilation: systematic review and meta-analysis. JAMA Intern. Med. 174 , 751–761 (2014).
de Smet, A. M. G. A. et al. Decontamination of the digestive tract and oropharynx in ICU patients. N. Engl. J. Med. 360 , 20–31 (2009).
Wittekamp, B. H. et al. Decontamination strategies and bloodstream infections with antibiotic-resistant microorganisms in ventilated patients: a randomized clinical trial. JAMA 320 , 2087–2098 (2018).
Dang, T. T., Eurich, D. T., Weir, D. L., Marrie, T. J. & Majumdar, S. R. Rates and risk factors for recurrent pneumonia in patients hospitalized with community-acquired pneumonia: population-based prospective cohort study with 5 years of follow-up. Clin. Infect. Dis. 59 , 74–80 (2014).
Garcia-Vidal, C. et al. Aetiology of, and risk factors for, recurrent community-acquired pneumonia. Clin. Microbiol. Infect. 15 , 1033–1038 (2009).
Liu, P. et al. Frequency of empiric antibiotic de-escalation in an acute care hospital with an established Antimicrobial Stewardship Program. BMC Infect. Dis. 16 , 751 (2016).
Maruyama, T. et al. A therapeutic strategy for all pneumonia patients: a 3-year prospective multicenter cohort study using risk factors for multidrug-resistant pathogens to select initial empiric therapy. Clin. Infect. Dis. 68 , 1080–1088 (2018).
Abdul-Aziz, M. H., Lipman, J. & Roberts, J. A. Antibiotic dosing for multidrug-resistant pathogen pneumonia. Curr. Opin. Infect. Dis. 30 , 231–239 (2017).
Tsai, D., Lipman, J. & Roberts, J. A. Pharmacokinetic/pharmacodynamic considerations for the optimization of antimicrobial delivery in the critically ill. Curr. Opin. Crit. Care 21 , 412–420 (2015).
Mandell, L. A. et al. Infectious Diseases Society of America/American Thoracic Society consensus guidelines on the management of community-acquired pneumonia in adults. Clin. Infect. Dis. 44 (Suppl 2), S27–S72 (2007).
Sligl, W. I. et al. Macrolides and mortality in critically ill patients with community-acquired pneumonia: a systematic review and meta-analysis. Crit. Care Med. 42 , 420–432 (2014).
Torres, A. et al. Challenges in severe community-acquired pneumonia: a point-of-view review. Intensive Care Med. 45 , 159–171 (2019).
Niederman, M. S. Macrolide-resistant pneumococcus in community-acquired pneumonia. Is there still a role for macrolide therapy? Am. J. Respir. Crit. Care Med. 191 , 1216–1217 (2015).
Asadi, L. et al. Guideline adherence and macrolides reduced mortality in outpatients with pneumonia. Respir. Med. 106 , 451–458 (2012).
Postma, D. F. et al. Cardiac events after macrolides or fluoroquinolones in patients hospitalized for community-acquired pneumonia: post-hoc analysis of a cluster-randomized trial. BMC Infect. Dis. 19 , 17 (2019).
Garin, N. et al. β-Lactam monotherapy vs β-lactam-macrolide combination treatment in moderately severe community-acquired pneumonia: a randomized noninferiority trial. JAMA Intern. Med. 174 , 1894–1901 (2014).
Gershengorn, H. B., Keene, A., Dzierba, A. L. & Wunsch, H. The association of antibiotic treatment regimen and hospital mortality in patients hospitalized with Legionella pneumonia. Clin. Infect. Dis. 60 , e66–e79 (2015).
Niederman, M. S. Antibiotic treatment of hospital-acquired pneumonia: is it different from ventilator-associated pneumonia? Curr. Opin. Crit. Care 24 , 353–360 (2018).
Kumar, A., Safdar, N., Kethireddy, S. & Chateau, D. A survival benefit of combination antibiotic therapy for serious infections associated with sepsis and septic shock is contingent only on the risk of death: a meta-analytic/meta-regression study. Crit. Care Med. 38 , 1651–1664 (2010).
Martin-Loeches, I. et al. Potentially resistant microorganisms in intubated patients with hospital-acquired pneumonia: the interaction of ecology, shock and risk factors. Intensive Care Med. 39 , 672–681 (2013).
Niederman, M. S. et al. Inhaled amikacin adjunctive to intravenous standard-of-care antibiotics in mechanically ventilated patients with Gram-negative pneumonia (INHALE): a double-blind, randomised, placebo-controlled, phase 3, superiority trial. Lancet Infect. Dis. 20 , 330–340 (2020).
Chastre, J. et al. Comparison of 8 vs 15 days of antibiotic therapy for ventilator-associated pneumonia in adults: a randomized trial. JAMA 290 , 2588–2598 (2003). A seminal article comparing 8 or 15 days of antibiotic treatment in VAP .
Garnacho-Montero, J. et al. Task force on management and prevention of Acinetobacter baumannii infections in the ICU. Intensive Care Med. 41 , 2057–2075 (2015).
Timsit, J.-F., Pilmis, B. & Zahar, J.-R. How should we treat hospital-acquired and ventilator-associated pneumonia caused by extended-spectrum β-lactamase-producing enterobacteriaceae? Semin. Respir. Crit. Care Med. 38 , 287–300 (2017).
de Jong, E. et al. Efficacy and safety of procalcitonin guidance in reducing the duration of antibiotic treatment in critically ill patients: a randomised, controlled, open-label trial. Lancet Infect. Dis. 16 , 819–827 (2016).
Sousa, D. et al. Community-acquired pneumonia in immunocompromised older patients: incidence, causative organisms and outcome. Clin. Microbiol. Infect. 19 , 187–192 (2013).
Ramirez, J. A. et al. Treatment of community-acquired pneumonia in immunocompromised adults: a consensus statement regarding initial strategies. Chest 158 , 1896–1911 (2020).
El-Solh, A. A. et al. Microbiology of severe aspiration pneumonia in institutionalized elderly. Am. J. Respir. Crit. Care Med. 167 , 1650–1654 (2003).
Siemieniuk, R. A. C. et al. Corticosteroid therapy for patients hospitalized with community-acquired pneumonia: a systematic review and meta-analysis. Ann. Intern. Med. 163 , 519–528 (2015).
Torres, A. et al. Effect of corticosteroids on treatment failure among hospitalized patients with severe community-acquired pneumonia and high inflammatory response: a randomized clinical trial. JAMA 313 , 677–686 (2015).
Rodrigo, C., Leonardi-Bee, J., Nguyen-Van-Tam, J. & Lim, W. S. Corticosteroids as adjunctive therapy in the treatment of influenza. Cochrane Database Syst. Rev. 3 , CD010406 (2016).
Recovery Collaborative Group. et al. Dexamethasone in hospitalized patients with Covid-19. N. Engl. J. Med. 384 , 693–704 (2021).
Welte, T. et al. Efficacy and safety of trimodulin, a novel polyclonal antibody preparation, in patients with severe community-acquired pneumonia: a randomized, placebo-controlled, double-blind, multicenter, phase II trial (CIGMA study). Intensive Care Med. 44 , 438–448 (2018).
Frat, J.-P. et al. High-flow oxygen through nasal cannula in acute hypoxemic respiratory failure. N. Engl. J. Med. 372 , 2185–2196 (2015).
Kaplan, V. et al. Pneumonia: still the old man’s friend? Arch. Intern. Med. 163 , 317–323 (2003).
Shorr, A. F. et al. Readmission following hospitalization for pneumonia: the impact of pneumonia type and its implication for hospitals. Clin. Infect. Dis. 57 , 362–367 (2013).
Chalmers, J. D. et al. Epidemiology, antibiotic therapy, and clinical outcomes in health care-associated pneumonia: a UK cohort study. Clin. Infect. Dis. 53 , 107–113 (2011).
El Moussaoui, R. et al. Long-term symptom recovery and health-related quality of life in patients with mild-to-moderate-severe community-acquired pneumonia. Chest 130 , 1165–1172 (2006).
Wootton, D. G. et al. A longitudinal modelling study estimates acute symptoms of community acquired pneumonia recover to baseline by 10 days. Eur. Respir. J. 49 , 1602170 (2017).
Marrie, T. J., Lau, C. Y., Wheeler, S. L., Wong, C. J. & Feagan, B. G. Predictors of symptom resolution in patients with community-acquired pneumonia. Clin. Infect. Dis. 31 , 1362–1367 (2000).
Almirall, J. et al. Epidemiology of community-acquired pneumonia in adults: a population-based study. Eur. Respir. J. 15 , 757–763 (2000).
Andrade, L. F. et al. Health related quality of life in patients with community-acquired pneumococcal pneumonia in France. Health Qual. Life Outcomes 16 , 28 (2018).
Wyrwich, K. W., Yu, H., Sato, R. & Powers, J. H. Observational longitudinal study of symptom burden and time for recovery from community-acquired pneumonia reported by older adults surveyed nationwide using the CAP Burden of Illness Questionnaire. Patient Relat. Outcome Meas. 6 , 215–223 (2015).
Carratala, J. et al. Outpatient care compared with hospitalization for community-acquired pneumonia: a randomized trial in low-risk patients. Ann. Intern. Med. 142 , 165–172 (2005).
Mangen, M.-J. J., Huijts, S. M., Bonten, M. J. M. & de Wit, G. A. The impact of community-acquired pneumonia on the health-related quality-of-life in elderly. BMC Infect. Dis. 17 , 208 (2017).
Lloyd, M., Callander, E., Karahalios, A., Desmond, L. & Karunajeewa, H. Patient-reported outcome measures in community-acquired pneumonia: a systematic review of application and content validity. BMJ Open. Respir. Res. 6 , e000398 (2019).
Gao, C. A. et al. Comparing nasopharyngeal and BAL SARS-CoV-2 assays in respiratory failure. Am. J. Respir. Crit. Care Med. 203 , 127–129 (2021).
Peiffer-Smadja, N. et al. Performance and impact of a multiplex PCR in ICU patients with ventilator-associated pneumonia or ventilated hospital-acquired pneumonia. Crit. Care 24 , 366 (2020).
Murphy, C. N. et al. Multicenter evaluation of the biofire filmarray pneumonia/pneumonia plus panel for detection and quantification of agents of lower respiratory tract infection. J. Clin. Microbiol. 58 , e00128-20 (2020).
Pendleton, K. M. et al. Rapid pathogen identification in bacterial pneumonia using real-time metagenomics. Am. J. Respir. Crit. Care Med. 196 , 1610–1612 (2017).
Chiu, C. Y. & Miller, S. A. Clinical metagenomics. Nat. Rev. Genet. 20 , 341–355 (2019).
Hellyer, T. P. et al. Biomarker-guided antibiotic stewardship in suspected ventilator-associated pneumonia (VAPrapid2): a randomised controlled trial and process evaluation. Lancet Respir. Med. 8 , 182–191 (2020).
Blot, S. I. et al. A clinical algorithm to diagnose invasive pulmonary aspergillosis in critically ill patients. Am. J. Respir. Crit. Care Med. 186 , 56–64 (2012).
Bassetti, M., Welte, T. & Wunderink, R. G. Treatment of Gram-negative pneumonia in the critical care setting: is the beta-lactam antibiotic backbone broken beyond repair? Crit. Care 20 , 19 (2016).
Kollef, M. H. et al. Ceftolozane-tazobactam versus meropenem for treatment of nosocomial pneumonia (ASPECT-NP): a randomised, controlled, double-blind, phase 3, non-inferiority trial. Lancet Infect. Dis. 19 , 1299–1311 (2019). A randomized clinical trial comparing ceftolozane–tazobactam with meropenem in ventilated HAP and VAP. A post-hoc analysis in ventilated HAP demonstrated superiority of ceftolozane–tazobactam .
Kollef, M. H. et al. A randomized trial of 7-day doripenem versus 10-day imipenem-cilastatin for ventilator-associated pneumonia. Crit. Care 16 , R218 (2012).
File, T. M. et al. FOCUS 1: a randomized, double-blinded, multicentre, phase III trial of the efficacy and safety of ceftaroline fosamil versus ceftriaxone in community-acquired pneumonia. J. Antimicrob. Chemother. 66 , iii19–iii32 (2011).
Biedenbach, D. J., Kazmierczak, K., Bouchillon, S. K., Sahm, D. F. & Bradford, P. A. In vitro activity of aztreonam-avibactam against a global collection of Gram-negative pathogens from 2012 and 2013. Antimicrob. Agents Chemother. 59 , 4239–4248 (2015).
Awad, S. S. et al. A phase 3 randomized double-blind comparison of ceftobiprole medocaril versus ceftazidime plus linezolid for the treatment of hospital-acquired pneumonia. Clin. Infect. Dis. 59 , 51–61 (2014).
David, S. et al. Epidemic of carbapenem-resistant Klebsiella pneumoniae in Europe is driven by nosocomial spread. Nat. Microbiol. 4 , 1919–1929 (2019).
Watkins, R. R. & File, T. M. Lefamulin: a novel semisynthetic pleuromutilin antibiotic for community-acquired bacterial pneumonia. Clin. Infect. Dis. 71 , 2757–2762 (2020).
Spellberg, B., Bartlett, J., Wunderink, R. & Gilbert, D. N. Novel approaches are needed to develop tomorrow’s antibacterial therapies. Am. J. Respir. Crit. Care Med. 191 , 135–140 (2015).
Matteo Bassetti, R. E. et al. Efficacy and safety of cefiderocol for the treatment of serious infections caused by carbapenem-resistant Gram-negative bacteria (CREDIBLE-CR): results of a phase 3 randomised, open-label, parallel-assigned, pathogen-focused study. Lancet 21 , 226–240 (2021).
Barnes, M. D. et al. Targeting multidrug-resistant Acinetobacter spp.: sulbactam and the diazabicyclooctenone β-lactamase inhibitor ETX2514 as a novel therapeutic agent. mBio 10 , e00159-19 (2019).
Lehman, K. M. & Grabowicz, M. Countering gram-negative antibiotic resistance: recent progress in disrupting the outer membrane with novel therapeutics. Antibiotics (Basel) 8 , 163 (2019).
Wu, J. Y., Srinivas, P. & Pogue, J. M. Cefiderocol: a novel agent for the management of multidrug-resistant gram-negative organisms. Infect. Dis. Ther. 9 , 17–40 (2020).
Wunderink, R. G. et al. Cefiderocol versus high-dose, extended-infusion meropenem for the treatment of Gram-negative nosocomial pneumonia (APEKS-NP): a phase 3, randomised, double-blind, non-inferiority study. Lancet Infect. Dis. 21 , 213–225 (2020).
File, T. M. et al. Efficacy and safety of IV-to-oral lefamulin, a pleuromutilin antibiotic, for treatment of community-acquired bacterial pneumonia: the phase 3 LEAP 1 trial. Clin. Infect. Dis. 69 , 1856–1867 (2019).
Alexander, E. et al. Oral lefamulin vs moxifloxacin for early clinical response among adults with community-acquired bacterial pneumonia: the LEAP 2 randomized clinical trial. JAMA 322 , 1661–1671 (2019).
Que, Y.-A. et al. Assessment of panobacumab as adjunctive immunotherapy for the treatment of nosocomial Pseudomonas aeruginosa pneumonia. Eur. J. Clin. Microbiol. Infect. Dis. 33 , 1861–1867 (2014).
François, B. et al. Safety and pharmacokinetics of an anti-PcrV PEGylated monoclonal antibody fragment in mechanically ventilated patients colonized with Pseudomonas aeruginosa: a randomized, double-blind, placebo-controlled trial. Crit. Care Med. 40 , 2320–2326 (2012).
François, B. et al. Safety and tolerability of a single administration of AR-301, a human monoclonal antibody, in ICU patients with severe pneumonia caused by Staphylococcus aureus: first-in-human trial. Intensive Care Med. 44 , 1787–1796 (2018).
Maddocks, S. et al. Bacteriophage therapy of ventilator-associated pneumonia and empyema caused by Pseudomonas aeruginosa. Am. J. Respir. Crit. Care Med. 200 , 1179–1181 (2019).
Wunderink, R. G. Turning the phage on treatment of antimicrobial-resistant pneumonia. Am. J. Respir. Crit. Care Med. 200 , 1081–1082 (2019).
Sicot, N. et al. Methicillin resistance is not a predictor of severity in community-acquired Staphylococcus aureus necrotizing pneumonia – results of a prospective observational study. Clin. Microbiol. Infect. 19 , E142–E148 (2013).
Download references
Acknowledgements
A.T. is the recipient of ICREA award from Generalitat de Catalunya. C.C. is the recipient of the SEPAR fellowship 2018, a grant 2019 from the Fondo de Investigación Sanitaria (PI19/00207), and the SEPAR fellowship “Programa Mentor”. We thank J.J.T.H. Roelofs (Department of Pathology, Amsterdam UMC, Amsterdam, Netherlands) for his invaluable assistance with the section on lung pathology and in providing representative histopathology slides.
Author information
Authors and affiliations.
Department of Pneumology, Hospital Clinic of Barcelona, Barcelona, Spain
Antoni Torres & Catia Cilloniz
August Pi i Sunyer Biomedical Research Institute (IDIBAPS), Barcelona, Spain
University of Barcelona, Barcelona, Spain
Biomedical Research Networking Centers in Respiratory Diseases (CIBERES and CIBERESUCICOVID study), Barcelona, Spain
Division of Pulmonary and Critical Care, New York Presbyterian/Weill Cornell Medical Center, New York City, NY, USA
Michael S. Niederman
Department of Pneumology, Hospital Universitario y Politécnico La Fe, Valencia, Spain
Rosario Menéndez
Scottish Centre for Respiratory Research, University of Dundee, Ninewells Hospital and Medical School, Dundee, UK
James D. Chalmers
Division of Pulmonary and Critical Care, Department of Medicine, Northwestern University Feinberg School of Medicine, Chicago, IL, USA
Richard G. Wunderink
Center of Experimental and Molecular Medicine, Division of Infectious Diseases, Amsterdam University Medical Centers, Location Academic Medical Center, University of Amsterdam, Amsterdam, Netherlands
Tom van der Poll
You can also search for this author in PubMed Google Scholar
Contributions
Introduction (C.C. and A.T.); Epidemiology (C.C. and R.M.); Mechanisms/pathophysiology (T.v.d.P.); Diagnosis, screening and prevention (C.C. and A.T.); Management (M.S.N.); Quality of life (J.D.C.); Outlook (R.G.W); Overview of Primer (A.T. and C.C.).
Corresponding authors
Correspondence to Antoni Torres or Catia Cilloniz .
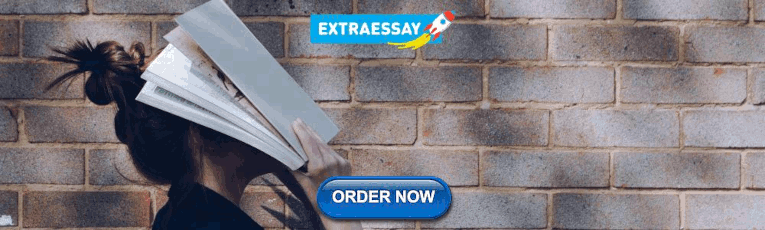
Ethics declarations
Competing interests.
A.T. has been a paid consultant to Pfizer, Jansen, and MSD, and a speaker for Pfizer and MSD. M.S.N. has received research grants from Shionogi, Bayer and Merck. He has been a paid consultant to Bayer, Merck, Paratek, Abbvie, Nabriva, and Thermo-Fisher. J.D.C. has received research funding from Astrazeneca, Boehringer-Ingelheim, Gilead Sciences, Glaxosmithkline, Insmed and Novartis; he has received consultancy fees from Chiesi, Grifols and Zambon. R.G.W. is a consultant to Merck, Shionogi, Polyphor, Microbiotix, bioMerieux, Curetis, KBP Biosciences, Idorsia and Accelerate. All other authors declare no competing interests.
Additional information
Peer review information.
Nature Reviews Disease Primers thanks Y. Arabi, C. Ginocchio, K. Klugman, M. Metersky, N. Suttorp and the other, anonymous, reviewer(s) for their contribution to the peer review of this work.
Publisher’s note
Springer Nature remains neutral with regard to jurisdictional claims in published maps and institutional affiliations.
Rights and permissions
Reprints and permissions
About this article
Cite this article.
Torres, A., Cilloniz, C., Niederman, M.S. et al. Pneumonia. Nat Rev Dis Primers 7 , 25 (2021). https://doi.org/10.1038/s41572-021-00259-0
Download citation
Accepted : 26 February 2021
Published : 08 April 2021
DOI : https://doi.org/10.1038/s41572-021-00259-0
Share this article
Anyone you share the following link with will be able to read this content:
Sorry, a shareable link is not currently available for this article.
Provided by the Springer Nature SharedIt content-sharing initiative
This article is cited by
Risk factors and predicting nomogram for the clinical deterioration of non-severe community-acquired pneumonia.
- Cheng-bin Xu
- Shan-shan Su
BMC Pulmonary Medicine (2024)
Validating the accuracy of deep learning for the diagnosis of pneumonia on chest x-ray against a robust multimodal reference diagnosis: a post hoc analysis of two prospective studies
- Jeremy Hofmeister
- Nicolas Garin
- Virginie Prendki
European Radiology Experimental (2024)
Hospitalization, case fatality, comorbidities, and isolated pathogens of adult inpatients with pneumonia from 2013 to 2022: a real-world study in Guangzhou, China
- Zhufeng Wang
- Jinping Zheng
BMC Infectious Diseases (2024)
A methodical exploration of imaging modalities from dataset to detection through machine learning paradigms in prominent lung disease diagnosis: a review
- Sunil Kumar
- Harish Kumar
- Manoj Diwakar
BMC Medical Imaging (2024)
Prediction of new-onset atrial fibrillation with the C2HEST score in patients admitted with community-acquired pneumonia
- Daniele Pastori
- Danilo Menichelli
- Roberto Cangemi
Infection (2024)
Quick links
- Explore articles by subject
- Guide to authors
- Editorial policies
Sign up for the Nature Briefing newsletter — what matters in science, free to your inbox daily.

- Case report
- Open access
- Published: 05 October 2018
Corynebacteria as a cause of pulmonary infection: a case series and literature review
- Katharine Yang 1 ,
- Robert L. Kruse 1 ,
- Weijie V. Lin 1 &
- Daniel M. Musher 1 , 2
Pneumonia volume 10 , Article number: 10 ( 2018 ) Cite this article
38k Accesses
31 Citations
26 Altmetric
Metrics details
In most cases of community-acquired pneumonia (CAP), an etiologic agent is not determined; the most common report from the microbiological evaluation of sputum cites “normal respiratory flora.” Non-diphtheria Corynebacterium spp. , a component of this flora, is commonly viewed as a contaminant, but it may be the cause of pneumonia and the frequency with which it causes CAP may be underestimated.
Case presentations
This report present 3 cases of CAP in which Corynebacterium spp. was clearly the predominant isolate; identification was confirmed by matrix-assisted laser desorption ionization time of flight (MALDI-TOF) mass spectrometry. Two cases were caused by C. propinquum and one by C. striatum. Two patients had a tracheostomy and one was on hemodialysis. Patients who received an appropriate antibiotic responded well.
When identified as the predominant isolate in sputum from a patient with CAP, Corynebacterium spp. should be considered as a potential cause of the infection. In cases with patients who have compromised airway clearance or who are immunocompromised, microaspiration may be responsible. While some Corynebacterium spp. are suspectible to antibiotics usually prescribed for CAP, others are susceptible only to vancomycin or aminoglycosides. Vancomycin is thus the appropriate empiric antibiotic, pending speciation and susceptibility test results. The number of reported cases with result of antibiotic susceptibility testing, however, remains limited, and further investigation is needed. Non-diphtheria Corynebacterium spp. represent a noteworthy clinical cause of pneumonia. Identification by Gram stain and as a predominant organism on culture demands careful consideration for management.
Pneumonia is an important cause of medical morbidity and mortality worldwide; pneumonia and influenza are listed as the 8th leading cause of death in the United States, with 57,062 deaths in 2015 [ 1 ]. Well-recognized and common causes of community-acquired pneumonia (CAP) in adults include bacteria such as Streptococcus pneumoniae, Haemophilus influenzae , and Staphylococcus aureus , and viruses such as influenza virus, respiratory syncytial virus, human metapneumovirus, and parainfluenza virus. Intensive recent investigations have failed to identify a causative organism in 50–62% of patients hospitalized for CAP [ 2 , 3 ].
The most common laboratory report for sputum samples submitted for microbiological study is “mixed respiratory flora.” Pneumonia caused by S. pneumoniae is thought to result from unrecognized microaspiration of pneumococci that have colonized the nasopharynx [ 4 ]. The authors of the present study have previously hypothesized that unrecognized aspiration of less virulent bacteria that normally inhabit the nasopharynx might also cause pneumonia, especially in persons whose upper airways are bypassed or whose ability to clear aspirated organisms is damaged [ 5 ]. The present study examined the possibilities that: (i) careful examination of Gram-stained sputum and culture plates may reveal a predominant bacterium such as Corynebacterium spp. that are not generally regarded as a pulmonary pathogen; (ii) quantitative cultures may document a high concentration of these bacteria in sputum; and (iii) these organisms are in fact the cause of some cases of pneumonia.
Corynebacterium spp. Gram-positive bacilli that are often dismissed as contaminants have been reported to cause pneumonia (Table 1 ). New technologies, such as matrix-assisted laser desorption ionization time of flight (MALDI-TOF) mass spectrometry enable rapid and precise identification of bacterial species [ 6 ], and highly sensitive techniques such as polymerase chain reaction (PCR) can help exclude a role for viruses, mycoplasma, and chlamydia. With the advent of these new technologies, there has been greater interest in Corynebacteria , and these organisms have been cited as emerging pathogens [ 7 ].
The present study reports on 3 well-documented cases of CAP due to Corynebacteria (2 cases of C. propinquum , 1 case of C. striatum ) that were diagnosed in a 6-month period at a large tertiary care hospital. The study reviews the medical literature on Corynebacterium spp. as etiologic agents of pneumonia, with the goal of increasing awareness of the possibility that so-called “normal respiratory flora” may actually be responsible for an as-yet undetermined proportion of cases of CAP.
A 75-year-old man on hemodialysis for end-stage renal disease was hospitalized for cough, fever, and altered mental status. His temperature was 103 °F, pulse 68, blood pressure 124/69, respiratory rate 22, and O 2 saturation 96% on 2 L of O 2 by nasal cannula. He was lethargic, with bibasilar crackles and a normal cardiac examination. His white blood cell (WBC) count was 20,100 cells/mm 3 and his plasma procalcitonin was 1.1 ng/mL. Chest X-ray showed bibasilar infiltrates. Microscopic examination of Gram-stained sputum showed profuse polymorphonuclear leukocytes (PMNs) and Gram-positive rods, many of which appeared to be intracellular (Fig. 1 ). Sputum culture yielded overwhelmingly predominant Corynebacteria , with rare P. aeruginosa . The Corynebacteria was identified as C. propinquum by MALDI-TOF. Blood cultures and viral PCR were negative. The patient was initially treated with vancomycin, cefepime, and metronidazole. Based on the predominance of Corynebacteria with the absence of other bacteria on Gram stain and a negative PCR for respiratory viruses, his pneumonia was attributed to C. propinquum , and only vancomycin was continued. He responded and was discharged to complete a 10-day course of linezolid.
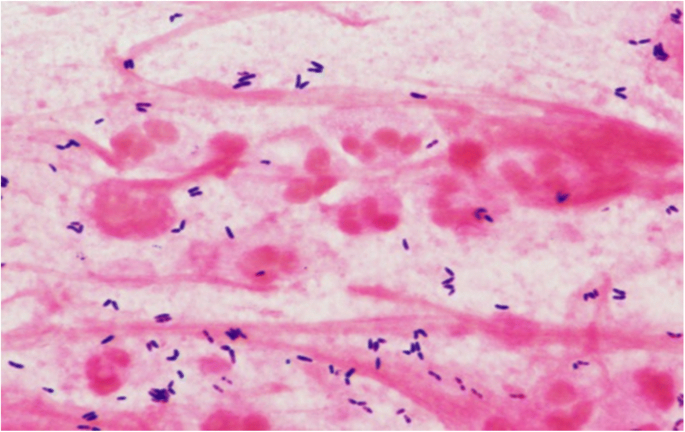
Gram-stain observed in sputum from patient with Corynebacterial pneumonia. A sputum sample collected from the patient in Case 1 depicts Gram-positive bacilli (purple) within and surrounding polymorphonuclear cells (pink). The “club-shaped” appearance pathognomonic for the group “Coryneform” is appreciated
A 61-year-old man was found unresponsive post laryngectomy and tracheostomy for laryngeal squamous cell carcinoma. His temperature was 97.8 °F, pulse 99, blood pressure 114/86, and respiratory rate 22. Breath sounds were decreased bilaterally. WBC count was 7200 cells/mm 3 , lactate 1.2 mg/dL. Chest X-ray showed a new left-sided infiltrate. Sputum Gram stain revealed many Gram-positive rods, including those found within PMNs. Sputum yielded many Corynebacteria, identified by MALDI-TOF as C. propinquum , and few S. aureus . Sputum was liquefied with 2% N-acetyl cysteine and diluted serially; aliquots were cultured on blood agar and the number of colony forming units (CFU) was calculated [ 8 ]. The specimen contained 2 × 10 9 CFU of Corynebacteria and < 10 5 CFU of S. aureus per mL. Blood cultures and viral PCR were negative. The patient was initially given vancomycin, cefepime, and ampicillin; treatment was switched to ampicillin/sulbactam after the Corynebacterium was reported susceptible. His mental status rapidly improved, and he was subsequently discharged to complete a 10-day course of amoxicillin/clavulanate.
A 59-year-old male with widely metastatic squamous cell carcinoma of the tongue was admitted for bleeding from his tracheostomy site. His temperature was 99 °F, blood pressure 108/84, pulse 119, respiratory rate 24, and oxygen saturation 82% on room air. He had blood at the tracheostomy site and bibasilar rhonchi. WBC count was 22,000 cells/mm 3 , hemoglobin 11.1 g/dL, and lactate 1.8 mg/dL. Chest X-ray revealed a left upper-lobe infiltrate. Sputum sample showed profuse PMNs and Gram-positive rods. Culture yielded C. striatum (confirmed by MALDI-TOF) and few Escherichia coli . The patient was placed on comfort care and died 8 days later.
Epidemiology
Non-diphtheria Corynebacterium spp.--commensal flora of the skin, respiratory tract, and mucous membranes [ 9 , 10 ]-- have been characterized as emerging pathogens [ 7 ]. Among reported cases, infections of the respiratory tract are second in frequency only to those of the urinary tract [ 11 ]. The literature search revealed 67 reported cases of pulmonary infection due to non-diphtheria Corynebacterium spp . , summarized in Table 1 . Cases include pneumonia (community-acquired, hospital-acquired, or ventilator-associated), necrotizing pneumonia, and empyema, although pneumonia predominated. Implicated Corynebacterium spp. include C. pseudodiphtheriticum [ 12 , 13 ], C. propinquum [ 14 ], C. striatum [ 14 , 15 , 16 , 17 , 18 ], C. afermentans [ 19 ], C. xerosis [ 20 ], C. jeikeium [ 10 , 21 , 22 , 23 ], C. pseudotuberculosis [ 24 , 25 ], C. mucifaciens [ 26 ], C. ulcerans [ 27 ], and C. macginleyi [ 28 ].
Microbiology, pathogenesis, and immunity
“Coryneform bacteria” is the umbrella term for the class of bacteria comprised of facultative intracellular, non-spore-forming, catalase-positive, non-acid-fast, non-motile, and irregularly-shaped Gram-positive rods [ 29 ]. Based on 16S rRNA sequencing studies, Corynebacterium belongs to a subdivision of Gram-positive eubacteria with high guanine-to-cytosine content, which also contains Arthrobacter, Mycobacterium, Nocardia , and Streptomyces [ 30 ]. Coryneform bacteria are pleomorphic throughout their life cycle, and may have thickenings at either end, giving the “coryneform” name, meaning “club-shaped”; they may be clustered, forming shapes that resemble a “V,” “palisades,” or “Chinese letters” [ 29 ]. Because of their relation to C. diphtheriae, their common name is “diphtheroids.”
Little is known about the virulence factors of the non-diphtheria Corynebacterium spp. that may contribute to CAP and other infections [ 31 ]. C. pseudodiphtheriticum, the species most commonly implicated as a cause of pneumonia, has an ability to invade human epithelial type 2 (HEp-2) cells and survive for 24 h after infection, suggesting a potential mechanism to escape killing by the innate immune system [ 31 ]. Invasive strains form biofilms and bind fibrinogen and fibronectin, which may be facilitated by fimbrial subunits [ 31 , 32 ].
Predisposing conditions
With the exception of 2 cases of pneumonia due to C. pseudotuberculosis in veterinary workers [ 24 , 25 ], all other reported cases of Corynebacterial pneumonia have occurred in persons whose upper airways are bypassed, whose ability to clear aspirated organisms is damaged, or who have immunocompromising conditions (Table 2 ). The great majority of patients have had conditions that affect pulmonary clearance, including chronic obstructive pulmonary disease (COPD), cystic fibrosis [ 33 ], and previous radiotherapy to the thorax [ 27 ]. Some have had procedures that bypass protective mechanisms of the upper airways such as recent endotracheal intubation [ 28 , 34 , 35 ] or laryngectomy [ 36 ], as seen in Cases 2 and 3 in the present study. Among patients who are immunocompromised, co-morbidities included HIV infection [ 17 , 37 , 38 ], chemotherapy [ 10 , 28 ], hematologic malignancies or conditions that compromise PMN function such as diabetes mellitus, alcohol use disorder [ 16 ] or end-stage renal disease, as seen in Case 1 in the present study.
Clinical description
The presentation of pneumonia due to Corynebacteria does not differ from that due to bacterial pneumonia of any other cause: cough, fever, and altered mental status are usually present, as observed in the present study’s 3 cases. Cases of community-acquired or ventilator-associated pneumonia, cavitary pneumonia, empyema, and bacteremia have been described (Table 1 ). Only 5 (7.5%) of the 67 reported patients with Corynebacterial pneumonia have been bacteremic with Corynebacteria. This suggests that blood cultures may be of minimal utility for confirming any sputum findings. Pneumonia caused by the quintessential pulmonary pathogen, S. pneumoniae, is bacteremic in no more than approximately 25% of cases [ 39 ], and the rate of bacteremia is far lower in pneumonia due to less pathogenic organisms, such as nontypable H. influenzae [ 40 ] or Moraxella catarrhalis [ 41 ].
Laboratory diagnosis
Given the limited utility of blood cultures in diagnosing Corynebacterial pneumonia, diagnosis requires a multifaceted approach. In most previous case reports, diagnosis has been based on microscopic examination of a Gram-stained sputum and careful examination of culture plates [ 42 ]. In the 3 cases discussed in this report, the sputum Gram stain showed profuse numbers of Corynebacteria . In 12 of the previously reported cases, quantitative studies were carried out on sputum, bronchoalveolar lavage samples, or protected bronchial brush samples (Table 1 ) with few or no colonies of other potentially pathogenic bacteria, as in Case 2 in which quantitative sputum culture revealed 1.2 × 10 9 CFU/mL. Pneumonia due to Corynebacteria may occur far more commonly than has been reported, because the finding of these organisms is usually dismissed as “normal mouth flora” or “normal respiratory flora.”
MALDI-TOF is useful in providing precise speciation, as was employed in the 3 cases discussed in this report. An analysis of 83 Corynebacterial samples compared MALDI-TOF with API Coryne V2.0 (bioMérieux, Marcy l’Etoile, France), which is the method that has been most widely used to speciate Corynebacteria in the past few decades. Identification matched in 73 of 83 (88%) samples [ 43 ]. 16S rRNA sequencing was used to resolve the discrepancy in the remaining 10 samples. All 10 matched with MALDI-TOF’s identification, which demonstrates the superiority of this methodology [ 43 , 44 ].
Corynebacterium spp. that have been identified as causes of pneumonia or empyema are delineated in Table 1 . C. pseudodiptheriticum, the cause of about two-thirds of reported Corynebacterial pneumonia cases, is susceptible to penicillin, cephalosporins, and vancomycin, but resistant to macrolides, clindamycin, trimethoprim/sulfamethoxazole, quinolones, and/or rifampin (Table 3 ). Some species, including C. jeikeium , C. macginleyi , and C. xerosis , are resistant to all common antibiotics except glycopeptides such as vancomycin (Table 3 ). Intermediately resistant organisms such as C. propinquum and C. striatum, implicated in the present study’s 3 cases, are also consistently susceptible to vancomycin and ampicillin, but variably susceptible to penicillin and cephalosporins, and commonly resistant to macrolides, clindamycin, trimethoprim-sulfamethoxazole, fluoroquinolines, and rifampin. The other reported Corynebacterium spp.— C. accolens, C. afermentans, C. mucifaciens, C. pseudotuberculosis, C. urealyticum, and C. ulcerans— had only 1–2 case reports each, and/or did not include comprehensive susceptibility reports. These cases are summarized in Table 3 . Although only one pneumonia case report [ 17 ] included susceptibility to linezolid or tigecycline, a prospective study analyzing infections due to Corynebacteria from a variety of sites revealed that all isolates—the majority being C. amycolatum, C. accolens, C. minutissimum, and C. glucuronolyticum (strains that are not regularly implicated in pneumonia)—were susceptible to linezolid and tigecycline [ 45 ].
These results suggest that, when a Gram-stained sputum from a patient with pneumonia reveals Corynebacteria as the predominant isolate, vancomycin should be the initial antibiotic of choice. If MALDI-TOF identifies a species that is likely to be susceptible to other antibiotics, the empiric antibiotic of choice can be revised, or definitive antibiotic treatment can be given based on results of antibiotic susceptibility testing as determined by gradient diffusion strip testing. There is no accepted recommendation for length of treatment for Corynebacterial pneumonia. However, among case reports, treatment time has ranged from 10 days for a case of C. macginleyi pneumonia [ 28 ], to 8 weeks for a cavitating pneumonia due to C. jeikeium [ 22 ], to 14 months for pneumonia due to C. pseudotuberculosis [ 25 ]. It seems reasonable to select a duration of treatment based on the nature of the lung lesion. Infiltrates should respond to the same 7 days of treatment that are used for other bacterial pneumonias, whereas cavitating pneumonia should be treated for longer periods, perhaps until the cavity is closed. In some cases, inadequate responses have been observed in cases of a lung abscess or cavitation, prompting surgical resection [ 16 , 37 ].
Conclusions
Although traditionally regarded as part of normal respiratory flora, Corynebacteria has the capacity to cause pneumonia in immunocompromised patients, or in immunocompetent patients who have impaired airway clearance and/or structural damage to the airways. The presentation is similar to that of any other bacterial pneumonia. Such infections may be far more common than has been previously suggested, because the finding of Corynebacteria on Gram stain and culture is likely to lead to a report of “mixed” or “normal respiratory flora.” A correct diagnosis can be made primarily by visualization of large numbers of diphtheroids in microscopic fields that contain > 20 WBC per epithelial cell, especially if the bacteria are located within PMNs, and supported by a culture in which the overwhelmingly predominant organism is Corynebacteria . Speciation by MALDI-TOF is increasingly available in clinical laboratories. Initial treatment should be with vancomycin. Speciation may guide antibiotic choice, but definitive therapy should be based on susceptibility testing using gradient diffusion strip testing methodology.
Abbreviations
Community-acquired pneumonia
Colony forming unit
Chronic obstructive pulmonary disease
Human epithelial type 2
Matrix-assisted laser desorption ionization– time of flight
Polymorphonuclear leukocytes
Ribosomal RNA
White blood cell
National Center for Health Statistics. Health, United States, 2016: With Chartbook on Long-term Trends in Health. Hyattsville, MD. 2017.
Musher DM, Roig IL, Cazares G, et al. Can an etiologic agent be identified in adults who are hospitalized for community-acquired pneumonia: results of a one-year study. J Inf Secur. 2013;67:11–8.
Google Scholar
Jain S, Self WH, Wunderink RG, Team CES. Community-acquired pneumonia requiring hospitalization. N Engl J Med. 2015;373:2382.
Article Google Scholar
Janoff EN, Musher DM. Streptococcus pneumoniae. In: Bennett JEDR, Blaser MJ, editors. Mandell, Douglas, and Bennett's principles and practice of infectious diseases. Philadelphia: Saunders; 2015. p. 2310–27.
Musher DM, Abers MS, Bartlett JG. Evolving understanding of the causes of pneumonia in adults, with special attention to the role of pneumococcus. Clin Infect Dis. 2017;65:1736–44.
Sloan A, Wang G, Cheng K. Traditional approaches versus mass spectrometry in bacterial identification and typing. Clin Chim Acta. 2017;473:180–5.
Article CAS Google Scholar
Harrington AT, Clarridge Iii JE, Mahlen SD. Chapter 91 - Corynebacterium spp. as established and emerging respiratory pathogens A2 - Tang, Yi-Wei. In: Sussman M, Liu D, Poxton I, Schwartzman J, editors. Molecular Medical Microbiology. 2nd ed. Boston: Academic Press; 2015. p. 1627–33.
Thorsteinsson SB, Musher DM, Fagan T. The diagnostic value of sputum culture in acute pneumonia. JAMA. 1975;233:894–5.
von Graevenitz A, Punter-Streit V, Riegel P, Funke G. Coryneform bacteria in throat cultures of healthy individuals. J Clin Microbiol. 1998;36:2087–8.
CAS PubMed PubMed Central Google Scholar
Waters BL. Pathology of culture-proven JK Corynebacterium pneumonia. An autopsy case report. Am J Clin Pathol. 1989;91:616–9.
Camello TC, Souza MC, Martins CA, et al. Corynebacterium pseudodiphtheriticum isolated from relevant clinical sites of infection: a human pathogen overlooked in emerging countries. Lett Appl Microbiol. 2009;48:458–64.
Ahmed K, Kawakami K, Watanabe K, et al. Corynebacterium pseudodiphtheriticum: a respiratory tract pathogen. Clin Infect Dis. 1995;20:41–6.
Manzella JP, Kellogg JA, Parsey KS. Corynebacterium pseudodiphtheriticum: a respiratory tract pathogen in adults. Clin Infect Dis. 1995;20:37–40.
Diez-Aguilar M, Ruiz-Garbajosa P, Fernandez-Olmos A, et al. Non-diphtheriae Corynebacterium species: an emerging respiratory pathogen. Eur J Clin Microbiol Infect Dis. 2013;32:769–72.
Creagh R, Saavedra JM, Rodriguez FJ, et al. Pneumonia casued by Corynebacterium striatum in a patient with AIDS. Enferm Infecc Microbiol Clin. 2000;18:297–8.
CAS PubMed Google Scholar
Martinez-Martinez L, Suarez AI, Ortega MC, Rodriguez-Jimenez R. Fatal pulmonary infection caused by Corynebacterium striatum. Clin Infect Dis. 1994;19:806–7.
Roig-Rico P, Safont-Gaso P, Marin-Tordera D, Ortiz-De la Tabla V. Corynebacterium striatum pneumonia in an HIV patient. Enferm Infecc Microbiol Clin. 2011;29:402.
Renom F, Gomila M, Garau M, et al. Respiratory infection by Corynebacterium striatum: epidemiological and clinical determinants. New Microbes New Infect. 2014;2:106–14.
Minkin R, Shapiro JM. Corynebacterium afermentans lung abscess and empyema in a patient with human immunodeficiency virus infection. South Med J. 2004;97:395–7.
Wallet F, Marquette CH, Courcol RJ. Multiresistant Corynebacterium xerosis as a cause of pneumonia in a patient with acute leukemia. Clin Infect Dis. 1994;18:845–6.
Ifantidou AM, Diamantidis MD, Tseliki G, et al. Corynebacterium jeikeium bacteremia in a hemodialyzed patient. Int J Infect Dis. 2010;14(Suppl 3):e265–8.
McNaughton RD, Villanueva RR, Donnelly R, et al. Cavitating pneumonia caused by Corynebacterium group JK. J Clin Microbiol. 1988;26:2216–7.
Yoshitomi Y, Kohno S, Koga H, et al. Fatal pneumonia caused by Corynebacterium group JK after treatment of Staphylococcus aureus pneumonia. Intern Med. 1992;31:930–2.
Keslin MH, McCoy EL, McCusker JJ, Lutch JS. Corynebacterium pseudotuberculosis. A new cause of infectious and eosinophilic pneumonia. Am J Med. 1979;67:228–31.
Heggelund L, Gaustad P, Havelsrud OE, et al. Corynebacterium pseudotuberculosis pneumonia in a veterinary student infected during laboratory work. Open Forum Infect Dis. 2015;2:ofv053.
Djossou F, Bezian MC, Moynet D, et al. Corynebacterium mucifaciens in an immunocompetent patient with cavitary pneumonia. BMC Infect Dis. 2010;10:355.
Siegel SM, Haile CA. Corynebacterium ulcerans pneumonia. South Med J. 1985;78:1267.
Kebbe J, Mador MJ. Corynebacterium macginleyi: a cause of ventilator associated pneumonia in an immunocompromised patient. Respir Med Case Rep. 2015;16:154–6.
PubMed PubMed Central Google Scholar
Funke G, von Graevenitz A, Clarridge JE 3rd, Bernard KA. Clinical microbiology of coryneform bacteria. Clin Microbiol Rev. 1997;10:125–59.
Woese CR. Bacterial evolution. Microbiol Rev. 1987;51:221–71.
Burkovski A. Corynebacterium pseudodiphtheriticum: putative probiotic, opportunistic infector, emerging pathogen. Virulence. 2015;6:673–4.
Souza MC, dos Santos LS, Sousa LP, et al. Biofilm formation and fibrinogen and fibronectin binding activities by Corynebacterium pseudodiphtheriticum invasive strains. Antonie Van Leeuwenhoek. 2015;107:1387–99.
Bittar F, Cassagne C, Bosdure E, et al. Outbreak of Corynebacterium pseudodiphtheriticum infection in cystic fibrosis patients, France. Emerg Infect Dis. 2010;16:1231–6.
Carranza Gonzalez R, Tena Gomez D, Prieto Gomez E, et al. Pneumonia by Corynebacterium pseudodiphteriticum: an infection to consider. An Med Interna. 2006;23:124–6.
Miller RA, Rompalo A, Coyle MB. Corynebacterium pseudodiphtheriticum pneumonia in an immunologically intact host. Diagn Microbiol Infect Dis. 1986;4:165–71.
Chiner E, Arriero JM, Signes-Costa J, et al. Corynebacterium pseudodiphtheriticum pneumonia in an immunocompetent patient. Monaldi Arch Chest Dis. 1999;54:325–7.
Gutierrez-Rodero F, Ortiz de la Tabla V, Martinez C, et al. Corynebacterium pseudodiphtheriticum: an easily missed respiratory pathogen in HIV-infected patients. Diagn Microbiol Infect Dis. 1999;33:209–16.
Roig P, Lopez MM, Arriero JM, et al. Corynebacterium pseudodiphtheriticum pneumonia in a patient diagnosed with HIV infection. An Med Interna. 1993;10:499–500.
Heffron R. Pneumonia with special reference to pneumococcus lobar pneumonia. New York: The Commonwealth Fund; 1939.
Musher DM, Kubitschek KR, Crennan J, Baughn RE. Pneumonia and acute febrile tracheobronchitis due to Haemophilus influenzae . Ann Intern Med. 1983;99:444–50.
Wallace RJ Jr, Musher DM. In honor of Dr. Sarah Branham, a star is born. The realization of Branhamella catarrhalis as a respiratory pathogen. Chest. 1986;90:447–50.
Coyle MB, Lipsky BA. Coryneform bacteria in infectious diseases: clinical and laboratory aspects. Clin Microbiol Rev. 1990;3:227–46.
Vila J, Juiz P, Salas C, et al. Identification of clinically relevant Corynebacterium spp., Arcanobacterium haemolyticum, and Rhodococcus equi by matrix-assisted laser desorption ionization-time of flight mass spectrometry. J Clin Microbiol. 2012;50:1745–7.
Bao R, Gao X, Hu B, Zhou Z. Matrix-assisted laser desorption ionization time-of-flight mass spectrometry: a powerful tool for identification of Corynebacterium species. J Thorac Dis. 2017;9:3239–45.
Reddy BS, Chaudhury A, Kalawat U, et al. Isolation, speciation, and antibiogram of clinically relevant non-diphtherial Corynebacteria (Diphtheroids). Indian J Med Microbiol. 2012;30:52–7.
Losada I, Daza RM, Merino J, et al. Corynebacterium CDC G1: pathogen or colonizer? Enferm Infecc Microbiol Clin. 1996;14:510–1.
Riebel W, Frantz N, Adelstein D, Spagnuolo PJ. Corynebacterium JK: a cause of nosocomial device-related infection. Rev Infect Dis. 1986;8:42–9.
Malkocoglu G, Gencer H, Kaya A, et al. Corynebacterium propinquum bronchopneumonia in a child with ataxia telangiectasia. Turk J Pediatr. 2016;58:558–61.
Furiasse D, Gasparotto AM, Monterisi A, et al. Pneumonia caused byCorynebacterium pseudodiphtheriticum. Rev Argent Microbiol. 2016;48:290–2.
PubMed Google Scholar
Chudnicka A, Szmygin-Milanowska K, Kieszko R, et al. The role of opportunistic species of Corynebacterium pseudodiphtheriticum in the pathogenesis of CAP (community acquired pneumonia). Ann Univ Mariae Curie Sklodowska Med. 2003;58:142–8.
Aspiroz Sancho C, Agustin Berne A, Navarro Pardos C, et al. Pneumonia caused by Corynebacterium pseudodiphteriticum, an entity worth knowing. An Med Interna. 2002;19:463–5.
Martaresche C, Fournier PE, Jacomo V, et al. A case of Corynebacterium pseudodiphtheriticum nosocomial pneumonia. Emerg Infect Dis. 1999;5:722–3.
Cohen Y, Force G, Gros I, et al. Corynebacterium pseudodiphtheriticum pulmonary infection in AIDS patients. Lancet. 1992;340:114–5.
Donaghy M, Cohen J. Pulmonary infection with Corynebacterium hofmannii complicating systemic lupus erythematosus. J Infect Dis. 1983;147:962.
Nishiyama A, Ishida T, Ito A, Arita M. Bronchopneumonia caused by Corynebacterium pseudodiphtheriticum. Intern Med. 2013;52:1847.
Cowling P, Hall L. Corynebacterium striatum: a clinically significant isolate from sputum in chronic obstructive airways disease. J Inf Secur. 1993;26:335–6.
CAS Google Scholar
Tarr PE, Stock F, Cooke RH, et al. Multidrug-resistant Corynebacterium striatum pneumonia in a heart transplant recipient. Transpl Infect Dis. 2003;5:53–8.
Verma R, Kravitz GR. Corynebacterium striatum empyema and osteomyelitis in a patient with advanced rheumatoid arthritis. BMJ Case Rep. 2016; https://doi.org/10.1136/bcr-2016-214691 .
Mattos-Guaraldi AL, Sampaio JL, Santos CS, et al. First detection of Corynebacterium ulcerans producing a diphtheria-like toxin in a case of human with pulmonary infection in the Rio de Janeiro metropolitan area, Brazil. Mem Inst Oswaldo Cruz. 2008;103:396–400.
Jacobs NF Jr, Perlino CA. “Diphtheroid” pneumonia. South Med J. 1979;72:475–6.
Nazemi MM, Musher DM. Empyema due to aerobic diphtheroids following dental extraction. Am Rev Respir Dis. 1973;108:1221–3.
Download references
Availability of data and materials
The data sets used and/or analyzed during the current study are available from the corresponding author on reasonable request.
Author information
Authors and affiliations.
Baylor College of Medicine, Houston, TX, 77030, USA
Katharine Yang, Robert L. Kruse, Weijie V. Lin & Daniel M. Musher
Infectious Disease Section, Michael E. DeBakey Veterans Affairs Medical Center, 2002 Holcombe Boulevard, Houston, TX, 77030, USA
Daniel M. Musher
You can also search for this author in PubMed Google Scholar
Contributions
KY tabulated previous literature. KY and DMM drafted the manuscript. All authors contributed to the design of the study and the writing of the manuscript. All authors read and approved the final manuscript.
Corresponding author
Correspondence to Daniel M. Musher .
Ethics declarations
Ethics approval and consent to participate.
Approval by the Institutional Review Board, Baylor College of Medicine.
Consent for publication
The data from these cases were collected under Protocol H-29468, approved by the Institution Review Board, Baylor College of Medicine. The protocol permits the study of patients admitted to the Michael E. DeBakey VA Medical Center with community acquired pneumonia for the purpose of reviewing etiology and appropriateness of treatment. The only image we show is a Gram stain of sputum which is a discarded sample. The cases that are reported have none of the 18 known patient identifiers.
Competing interests
The authors declare that they have no competing interests.
Publisher’s Note
Springer Nature remains neutral with regard to jurisdictional claims in published maps and institutional affiliations.
Rights and permissions
Open Access This article is distributed under the terms of the Creative Commons Attribution 4.0 International License ( http://creativecommons.org/licenses/by/4.0/ ), which permits unrestricted use, distribution, and reproduction in any medium, provided you give appropriate credit to the original author(s) and the source, provide a link to the Creative Commons license, and indicate if changes were made. The Creative Commons Public Domain Dedication waiver ( http://creativecommons.org/publicdomain/zero/1.0/ ) applies to the data made available in this article, unless otherwise stated.
Reprints and permissions
About this article
Cite this article.
Yang, K., Kruse, R.L., Lin, W.V. et al. Corynebacteria as a cause of pulmonary infection: a case series and literature review. Pneumonia 10 , 10 (2018). https://doi.org/10.1186/s41479-018-0054-5
Download citation
Received : 27 April 2018
Accepted : 18 September 2018
Published : 05 October 2018
DOI : https://doi.org/10.1186/s41479-018-0054-5
Share this article
Anyone you share the following link with will be able to read this content:
Sorry, a shareable link is not currently available for this article.
Provided by the Springer Nature SharedIt content-sharing initiative
- Corynebacteria
- Diphtheroids
- Normal respiratory flora
ISSN: 2200-6133
- General enquiries: [email protected]
Case report
- Open access
- Published: 16 March 2022
Atypical presentation of an atypical pneumonia: a case report
- Alvin Oliver Payus ORCID: orcid.org/0000-0003-4675-103X 1 ,
- Clarita Clarence 2 ,
- Tiong Nee 3 &
- Wan Nur Nafisah Wan Yahya 2
Journal of Medical Case Reports volume 16 , Article number: 105 ( 2022 ) Cite this article
2846 Accesses
1 Citations
1 Altmetric
Metrics details
Neurologic impediments occur in only 0.1% of Mycoplasma pneumoniae infections. Although direct intracerebral infection can occur in these patients, autoimmune-mediated reactions secondary to molecular mimicry are the most common pathophysiology of such neurological complications. These complications include immune-mediated encephalitis, peripheral neuritis such as Guillain–Barré syndrome, and many others. Miller Fisher syndrome is a one of the variants of Guillain–Barré syndrome that has been rarely linked to Mycoplasma pneumoniae infection. It is a condition classically characterized by the triad of ophthalmoplegia, areflexia, and ataxia. Most patients with Miller Fisher syndrome will have positive anti-ganglioside GQ1b antibodies found in their serum, making this autoantibody a very useful serological confirmation parameter. We report a case of a Miller Fisher syndrome in a woman with Mycoplasma pneumoniae infection. To the best of the authors’ knowledge, such cases have been only rarely described in literature.
Case presentation
A 35-year-old Chinese woman presented with sudden onset of double vision and ataxia 5 days after fever and mild flu symptoms. Her Mycoplasma pneumoniae antigen was positive with 1 over 2560 titer of total mycoplasma antibody and presence of immunoglobulin M antibody, suggesting acute infection, and her nerve conduction study revealed mild sensory axonal polyneuropathy with segmental demyelination. the Miller Fischer syndrome variant of Guillain-Barré syndrome secondary to Mycoplasma pneumonia was suspected and later confirmed by presence of serum anti-GQ1b autoantibody. She was treated with intravenous immunoglobulin 0.4 g/kg once daily for 5 days.
Conclusions
The objective of this report is to share a case of an uncommon neurological complication of Mycoplasma pneumoniae infection, to increase the level of suspicion among clinicians that Miller Fischer syndrome can occur as an atypical presentation of an atypical pneumonia.
Peer Review reports
Introduction
Mycoplasma pneumoniae (MP) is an atypical microorganism that commonly causes community-acquired pneumonia. This organism has peculiar properties that not only make it invisible on the usual Gram stain but also nonsusceptible to the broad-spectrum beta-lactam drugs usually applied as first-line antibiotics to treat community-acquired infection [ 1 ]. MP causes atypical pneumonia, associated with a list of extrapulmonary manifestations. These include hemolytic anemia, myringitis, Guillain–Barré syndrome and its variant, and many others. Miller Fisher syndrome is one of the rare extrapulmonary manifestations of MP infection [ 2 ]. This atypical presentation of an atypical pneumonia is the center of discussion in this case report.
A 35-year-old Chinese female with no known medical illness presented with double vision and body imbalances for the past 2 days. She described that the diplopia was of sudden onset, painless, and did not occur on looking in any specific direction. Regarding the body imbalance, she showed a tendency to sway to the right side. On further questioning, she also had history of preceding fever with mild flu symptoms for the past 5 days.
She also complained of numbness and cramping sensation over the hands and feet bilaterally, 1 day before presentation. Otherwise, there was no neck stiffness, no upper and lower limb weakness, no slurring of speech, no dysphagia, no dyspnea, and no history of recent accident or trauma. Upon arrival to the emergency department, she required assistance for ambulation as she has difficulty in maintaining balance. Her vital signs were stable with blood pressure of 124/78 mmHg, pulse rate of 80 beats per minute, regular rhythm and no collapsing character, afebrile, and not tachypneic. On physical examination, she had diplopia over the lateral gaze bilaterally, but otherwise there was no nystagmus, no dysdiadochokinesia, no dysmetria, and no facial weakness, and examination of the rest of the cranial nerves, upper limbs, and lower limbs revealed no abnormal findings. Romberg’s test was negative, but sharpened Romberg’s test was positive. Her abdomen was soft, not tender, and there was no palpable mass or organomegaly. Examination of the cardiorespiratory system revealed no abnormal findings. Initial blood investigation was normal (Table 1 ).
Blood investigations taken upon arrival to the hospital showed normal cell counts. There was no electrolyte abnormality, and the renal profile and liver function were also normal.
Computed tomographic scan of the brain revealed no intracranial bleeding and no space-occupying lesion (Fig. 1 ). Nerve conduction study revealed mild sensory axonal polyneuropathy with segmental demyelination. H reflex was absent bilaterally. Lumbar puncture for cerebrospinal fluid analysis was done and showed normal cell counts and biochemistry profile and no abnormal cells, and was negative for bacterial culture, multiple viral antibody panels, and cryptococcal antigen test. In view of the presence of ataxia and diplopia, the Miller Fischer syndrome variant of Guillain–Barré syndrome was suspected and later confirmed with the presence of serum anti-GQ1b autoantibody. Intravenous immunoglobulin (IVIg) 0.4 gm/kg once daily was started and planned to complete for 5 days. On the second day of admission, she developed worsening cough and shortness of breath. Radiographic imaging of the chest revealed homogeneous opacity over the lower right zone (Fig. 2 ). She was initially started on intravenous (IV) co-amoxiclav 1.2 g three times daily, and was escalated to IV piperacillin–tazobactam 4.5 gm three times daily after 2 days after she failed to show any improvement. As atypical pneumonia was suspected by the treating physician in view of the associated neurological symptoms, Mycoplasma pneumoniae antigen was taken and came back positive with 1 over 2560 titer of total mycoplasma antibody and presence of IgM antibody, suggestive of an acute infection. She was started with macrolide antibiotic (oral azithromycin 500 mg once daily for 5 days) on top of the previous IV antibiotic and IVIg treatment. She was also subjected to inpatient physiotherapy for a few days, then discharged well.
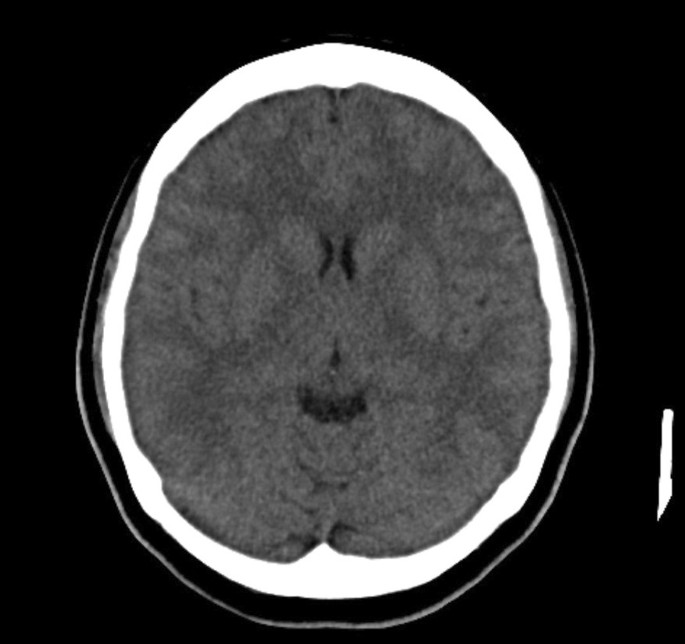
Computed tomographic imaging of brain on admission, showing no intracranial bleeding or space-occupying lesion
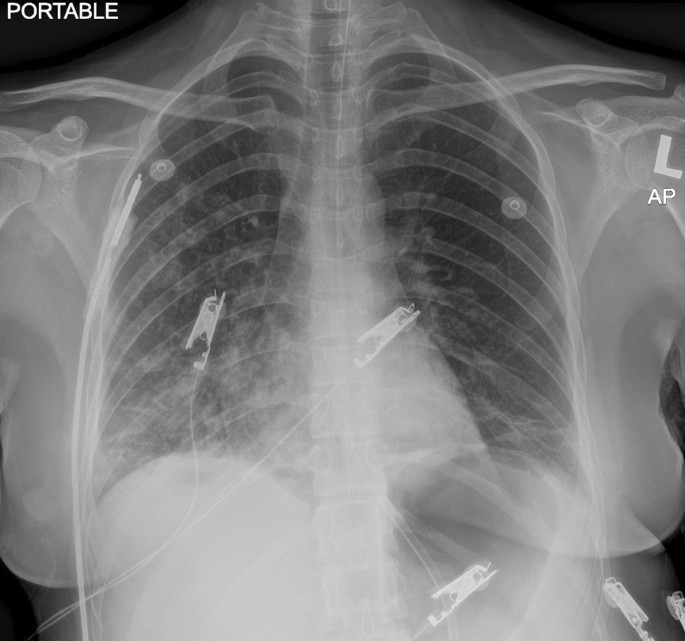
Radiographic imaging of chest taken on second day of admission when the patient developed shortness of breath, showing heterogeneous opacity over the lower right zone
Mycoplasma pneumoniae (MP) is a common respiratory pathogen. This short, rod-shaped bacterium lacking a cell wall commonly causes respiratory infections such as bronchitis and pneumonia. It is often considered to be an atypical organism as it is not visible on the usual Gram stain and cannot be cultured using standard methods [ 1 ]. This organism is also not susceptible to the broad-spectrum beta-lactam antibiotics normally used as first-line treatment for community-acquired pneumonia. MP is one of the causative organisms of “atypical” bacterial pneumonia because the respiratory manifestations are predominated by a complex of constitutional symptoms such as low-grade fever, headache, and malaise, usually associated with extrapulmonary manifestations such as hemolytic anemia, myocarditis, myringitis, encephalitis, and many others that can occur alongside or independent of the respiratory symptoms [ 2 ]. Miller Fisher syndrome (MFS) is one of the rare extrapulmonary manifestations of MP infection.
MFS is considered a rare variant of Guillain–Barré syndrome, which is an acute idiopathic, immune-mediated inflammatory polyradiculopathy. The pathophysiology of this condition is still not well understood, but autoantibody-mediated neuritis, a condition in which the immune system attacks the nerves as a result of molecular mimicry that can be triggered by various agents, is a possibility. Among the common pathogens are Campylobacter jejuni , Haemophilus influenzae , and cytomegalovirus. However, MP is a rare pathogen also found to be associated with MFS. MFS classically presents with a triad of ataxia, ophthalmoplegia, and areflexia. However, the clinical manifestations of MFS can vary widely. The less common presentations include limb dysesthesia, ptosis, facial and bulbar palsies, mild muscle weakness, and urinary incontinence [ 3 ]. MFS is two times more common in men and can affect people of all ages, with median age of onset in the fifth decade [ 4 ].
The diagnosis of MFS is made clinically. However, serological confirmation with the presence of Anti-GQ1b antibodies is often made to support the diagnosis. This antibody acts on GQ1b, which is a ganglioside found abundantly in the paranodal region of the extramedullary portion of the oculomotor, abducens, and trochlear nerves. These areas are usually those most affected by self-reactive Anti-GQ1b antibodies, blocking release of acetylcholine from the motor nerve ending [ 4 ]. Although this antibody is not unique to MFS, it can help support the diagnosis in cases of uncertainty. Moreover, it can also determine the severity of the disease, as the level relates to the disease activity.
MFS is a self-limiting condition, and gradual improvement marks its recovery period and often the resolution of symptoms. Rarely, serious complications such as cardiac arrhythmia or respiratory failure have been reported [ 5 ]. Ataxia and ophthalmoplegia typically resolve within 1–3 months after onset, and near-complete recovery is expected within 6 months [ 5 ]. Though areflexia may persist, it is not associated with functional disability. Although MFS follows a self-limiting course, immunomodulatory therapies including intravenous immune globulin and plasmapheresis have been used to hasten disease recovery and perhaps decrease the likelihood of progression to more severe conditions such as GBS [ 6 ]. Based on the observational data available, complete resolution of ataxia in 1 month and resolution of ophthalmoplegia within 3 months would be acceptable outcome measures [ 5 ].
In this report, our patient presented initially with ataxia and diplopia, suggestive of Miller Fischer syndrome that was later confirmed with the presence of anti-GQ1b antibody in serum. She was then treated with IVIg 0.4 g/kg once daily for a total of 5 days. However, she developed worsening respiratory symptoms later on during inpatient immunoglobulin therapy. She did not respond to first-line beta-lactam antibiotics and was investigated for atypical pneumonia, which was later confirmed with the presence of Mycoplasma pneumoniae antibody titer in serum and clinically response to macrolide antibiotic. She recovered well and is currently under regular outpatient clinic and physiotherapy follow-up.
The aim of this case report is to increase the level of suspicion among clinicians regarding the possibility of an association between Miller Fischer syndrome and Mycoplasma pneumoniae infection, which should be considered in any cases of pneumonia that develop neurological manifestations such as ophthalmoplegia and ataxia.
Availability of data and materials
Not applicable.
Baum SG. Mycoplasma pneumoniae infection in adults. UpToDate, Basow, DS (Ed), UpToDate, Waltham, MA. 2012.
Ran Nir-Paz. Atypical pneumonia. BMJ Best practice. Dec 2019.
Lo YL. Clinical and immunological spectrum of the Miller Fisher syndrome. Muscle Nerve. 2007;36(5):615–27.
Article CAS Google Scholar
Sumera B, Taboada J. A case of Miller Fisher syndrome and literature review. Cureus. 2017;9(2).
Gupta SK, Jha KK, Chalati MD, Alashi LT. Miller Fisher syndrome. Case Reports. 2016;13(2016):2016217085.
Google Scholar
Yepishin IV, Allison RZ, Kaminskas DA, Zagorski NM, Liow KK. Miller Fisher syndrome: a case report highlighting heterogeneity of clinical features and focused differential diagnosis. Hawai’i J Med Public Health. 2016;75(7):196.
Download references
Acknowledgements
The authors would like to thank the Director General of Ministry of Health of Malaysia for his permission to publish this article.
No funding was received for the writing of this article.
Author information
Authors and affiliations.
Medicine Based Department, Faculty of Medicine and Health Science, Universiti Malaysia Sabah (UMS), Jalan UMS, 88400, Kota Kinabalu, Sabah, Malaysia
Alvin Oliver Payus
Department of Internal Medicine, Universiti Kebangsaan Malaysia Medical Centre (UKMMC), Jalan Yaacob Latif, Cheras, 56000, Kuala Lumpur, Malaysia
Clarita Clarence & Wan Nur Nafisah Wan Yahya
Hospital Rehabilitasi Cheras, Jalan Yaacob Latif, Cheras, 56000, Kuala Lumpur, Malaysia
You can also search for this author in PubMed Google Scholar
Contributions
AOP was a major contributor to the writing of this manuscript. CC analyzed and interpreted the patient data. RH helped in data collection and contributed to writing the manuscript. WNNWY is the advisor and helped with writing and final editing of the manuscript. All authors read and approved the final manuscript
Corresponding author
Correspondence to Alvin Oliver Payus .
Ethics declarations
Ethics approval and consent to participate, consent for publication.
Written informed consent was obtained from the patient for publication of this case report and any accompanying images. A copy of the written consent is available for review by the Editor-in-Chief of this journal.
Competing interests
The authors declare that they have no competing interests.
Additional information
Publisher’s note.
Springer Nature remains neutral with regard to jurisdictional claims in published maps and institutional affiliations.
Rights and permissions
Open Access This article is licensed under a Creative Commons Attribution 4.0 International License, which permits use, sharing, adaptation, distribution and reproduction in any medium or format, as long as you give appropriate credit to the original author(s) and the source, provide a link to the Creative Commons licence, and indicate if changes were made. The images or other third party material in this article are included in the article's Creative Commons licence, unless indicated otherwise in a credit line to the material. If material is not included in the article's Creative Commons licence and your intended use is not permitted by statutory regulation or exceeds the permitted use, you will need to obtain permission directly from the copyright holder. To view a copy of this licence, visit http://creativecommons.org/licenses/by/4.0/ . The Creative Commons Public Domain Dedication waiver ( http://creativecommons.org/publicdomain/zero/1.0/ ) applies to the data made available in this article, unless otherwise stated in a credit line to the data.
Reprints and permissions
About this article
Cite this article.
Payus, A.O., Clarence, C., Nee, T. et al. Atypical presentation of an atypical pneumonia: a case report. J Med Case Reports 16 , 105 (2022). https://doi.org/10.1186/s13256-022-03320-y
Download citation
Received : 05 November 2021
Accepted : 09 February 2022
Published : 16 March 2022
DOI : https://doi.org/10.1186/s13256-022-03320-y
Share this article
Anyone you share the following link with will be able to read this content:
Sorry, a shareable link is not currently available for this article.
Provided by the Springer Nature SharedIt content-sharing initiative
- Miller Fisher syndrome
- Mycoplasma pneumoniae
- Molecular mimicry
- GQ1b ganglioside
Journal of Medical Case Reports
ISSN: 1752-1947
- Submission enquiries: Access here and click Contact Us
- General enquiries: [email protected]
We have a new app!
Take the Access library with you wherever you go—easy access to books, videos, images, podcasts, personalized features, and more.
Download the Access App here: iOS and Android . Learn more here!
- Remote Access
- Save figures into PowerPoint
- Download tables as PDFs

5: Community-Acquired Pneumonia
Sean N. Avedissian; Marc H. Scheetz
- Download Chapter PDF
Disclaimer: These citations have been automatically generated based on the information we have and it may not be 100% accurate. Please consult the latest official manual style if you have any questions regarding the format accuracy.
Download citation file:
- Search Book
Jump to a Section
Patient presentation.
- Full Chapter
- Supplementary Content
Chief Complaint
“I have been coughing, have chest pain, and cannot breathe for about 2 days now.”
History of Present Illness
WA is a 40-year-old Caucasian male who presents to the emergency department with a fever, cough, chest pain (worsening when breathing or coughing), and shortness of breath. Normally, he has a fairly active lifestyle as he trains for marathons. He has not traveled outside the United States recently. He states he has “been taking cough medicine at night” for the past 4 days to help him sleep, but it has not been getting better. Also, he reports that he has been waking up at night due to heavy sweating. He states all his symptoms have gotten worse in the last 2 days. After being assessed in the ED, WA is admitted to the medicine unit for further workup.
Past Medical History
Diabetes: Type 1
Surgical History
Family history.
Father has diabetes: Type 1, history of heart attack; mother has hypertension.
Social History
Married with no kids. Denies smoking and drinks alcohol occasionally (weekends, social events)
Home Medications
Insulin (bolus/basal: ~35 units total daily)
Aspirin 81 mg PO daily (cardiovascular protection)
Atorvastatin 20 mg PO daily (cardiovascular protection)
Physical Examination
Vital signs.
Temp 100.8°F, HR 110 bpm, RR 30, BP 125/75 mm Hg, p02 93%, Ht 5′9″, Wt 70 kg
Slightly lethargic, mild—moderate distress
Normocephalic, atraumatic, PERRLA, EOMI, normal mucus membranes and conjunctiva, adequate dentition
Diminished breath sounds and crackles (rales) bilaterally
Cardiovascular
NSR, no m/r/g
Soft, non-distended, non-tender, bowel sounds hyperactive
Lethargic, oriented to place and person, (–) Brudzinski’s sign, (–) Kernig’s sign
Extremities
No significant findings
States he cannot remember all of them. He says he received all his age-related vaccines when younger. Has not received his flu-shot this year as he always forgets to receive it.
Laboratory Findings
Sign in or create a free Access profile below to access even more exclusive content.
With an Access profile, you can save and manage favorites from your personal dashboard, complete case quizzes, review Q&A, and take these feature on the go with our Access app.
Pop-up div Successfully Displayed
This div only appears when the trigger link is hovered over. Otherwise it is hidden from view.
Please Wait
December 19, 2023
The Real Story Behind ‘White Lung Pneumonia’
Separate outbreaks of pneumonia in children have cropped up in the U.S., China and Europe. Public health experts say the uptick in cases is not caused by a novel pathogen
By Tara Haelle
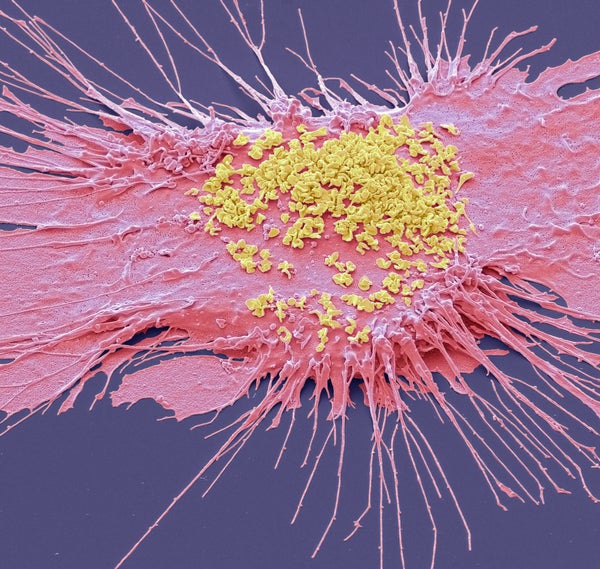
Colored scanning electron micrograph (SEM) of a dendritic cell infected with Mycoplasma pneumoniae (yellow). Mycoplasmas are bacteria that lack a cell wall. Without a cell wall, they are unaffected by many common antibiotics such as penicillin or other beta-lactam antibiotics that target cell wall synthesis.
Steve Gschmeissner/Science Source
Experts say a pneumonia outbreak among children in Ohio and a cluster of pneumonia cases in China are unrelated, despite some social media posts and tabloid articles that have ambiguously linked the two.
The usual respiratory pathogens are making their rounds this cold and flu season, yet the specter of the pandemic has left many on alert for the next novel agent.
“I understand outbreaks in China can make people nervous, but this is not that,” says Paul Offit , an infectious disease physician at Children’s Hospital of Philadelphia. Although another global illness may emerge in the future, the current pneumonia reports are “nothing to worry about,” he adds.
On supporting science journalism
If you're enjoying this article, consider supporting our award-winning journalism by subscribing . By purchasing a subscription you are helping to ensure the future of impactful stories about the discoveries and ideas shaping our world today.
Many pathogens that circulate in the Northern Hemisphere’s winter and year-round—including flu, respiratory syncytial virus (RSV) and now COVID—are far from benign and can lead to pneumonia in some cases. But experts say there is no reason to panic or interpret the current uptick in illnesses as anything other than the typical circulation of respiratory viruses and bacteria.
These are “just everyday pathogens that normally increase during the winter having a somewhat early and very assertive increase at the present time,” says William Schaffner , an infectious disease physician and a professor at Vanderbilt University Medical Center. But people are not helpless against these germs, says Rama Thyagarajan , an infectious disease and internal medicine physician at the University of Texas at Austin Dell Medical School. COVID, the flu and RSV all have vaccines that can reduce the risk of pneumonia, she says.
Schaffner agrees, calling these immunizations “the best present you can give in this holiday season to yourself and to your family and to your neighbors.”
Here’s what to know about the recent reports of pneumonia and the term “white lung pneumonia,” which has been used in some news coverage to describe the uptick in cases.
What clusters of pneumonia cases are being reported?
Warren County, Ohio’s public health department, which serves the northeastern suburbs of Cincinnati, reported a large uptick in the number of typical pneumonia cases in children, with 145 cases in those three to 14 years old recorded as of November 29. Massachusetts has also reported an increase in RSV and “walking pneumonia” among children.
Earlier in November China had reported an increase in respiratory disease cases. Chinese health officials attributed this uptick to the lift of COVID restrictions and the usual rise in known pathogens that can also make people vulnerable to pneumonia, including flu, COVID, RSV and infections caused by the common bacterium Mycoplasma pneumoniae . The World Health Organization is monitoring those cases, as well as an increase in pediatric respiratory disease cases in northern China.
Meanwhile multiple countries in Europe have also reported a rise in pediatric pneumonia cases, many of which are also caused by Mycoplasma bacteria.
None of these clusters, however, appear to be related to one another or caused by unfamiliar bugs. Initial reports on the increase in Warren County came from school nurses who said that a lot of students were calling in sick, according to Clint Koenig, a family physician and the medical director of Warren County Health District.
“We’re pretty confident that this is way above what we’ve seen this time last year,” he says. But Koenig adds that it’s hard to say how many more cases there are because data on children’s pneumonia cases are not routinely collected. Regardless, the causes of pneumonia are no different than those of past years: mostly RSV, adenovirus and Streptococcus or Mycoplasma infections.
What’s causing the current uptick in pneumonia cases, and how severe are they?
The growing pockets of pneumonia trace back to the usual increase in respiratory illnesses that occurs every winter, Schaffner says. Just as some flu seasons are more intense than others, the spread and severity of other winter diseases can also vary from year to year. Some upticks might be occurring as the usual seasonality of these pathogens continues to settle back into prepandemic patterns after it was disrupted by lockdowns, masking and social distancing, Schaffner says. But the biggest cause is likely that pathogens have more opportunity to spread in the winter.
“These viruses are taking advantage of us now that we are close together in birthday parties, schools, travel, religious services—whatever brings people together indoors,” Schaffner says. “And of course, we anticipate even more of that, given the holiday season. The New Year’s parties, all the travel associated with that and vacations are all wonderful environments that predispose to the spread of all of these respiratory infections, some of which will eventuate in pneumonia.”
What is the difference between pneumonia, “walking pneumonia” and “white lung syndrome”?
Pneumonia is an inflammation of the lungs that can be caused by a wide range of viruses, bacteria and fungi . Most respiratory infections involve the upper respiratory tract—the nose, throat and upper bronchial tubes, Schaffner says.
An infection develops into pneumonia when it reaches the lower respiratory tract and invades the lung tissue. This causes the lung’s white blood cells to trigger an inflammatory response. “If you get a lot of pneumonia, it will materially interfere with your ability to exchange gases. You can get short of breath, and you can have difficulty breathing,” Schaffner says.
Other symptoms include cough, fever, chest pain, fatigue and loss of appetite.
At least a dozen different pathogens can lead to pneumonia—no individual pathogen is responsible for even one in 10 cases . In fact, the pathogen behind any particular case of pneumonia is often never identified. Most pneumonia cases are triggered by a bacterium, but pneumonia is also a possible complication of respiratory viruses, such as COVID, influenza, RSV and even the common cold. These viruses can cause pneumonia by themselves or by making the body more vulnerable to secondary infections.
“Once somebody is infected with a virus, they’re more prone to get a bacterial infection on top of that” because the viral infection reduces their immune defenses, Thyagarajan explains. “The people that are affected are very young—infants and very young children—and very old and people with chronic illness.”
“Walking pneumonia” is a lay term often used to describe mild pneumonia cases, particularly those caused by Mycoplasma bacteria. It also has been called atypical pneumonia, Thyagarajan says, and can cause fevers, a dry cough and sometimes ear infections. According to Offit, “walking pneumonia” is usually not that severe. “Although we treat it with antibiotics, it usually is, for the most part, limited,” he says.
“White lung disease,” or “white lung syndrome,” is nothing but “a scary lay description, not used by medical professionals, of what we see on a routine chest x-ray,” Schaffner says. Healthy lungs full of air appear black in an x-ray because air looks dark in a normal reading. When inflammation and white blood cells fill the area, the lungs become opaque and more white on the reading, Offit explains. “It’s neither a scientific nor a medically acceptable term,” he adds.
How does pneumonia differ between children and adults?
Pneumonia symptoms are similar in children and adults, though young children may also experience nausea and vomiting, and older adults may have confusion. Beyond that, “different bugs are more apt to produce pneumonia in children than adults,” Schaffner says. “The older you get, if you have underlying illnesses, these respiratory viruses are more likely to result in pneumonia.”
Older adults tend to fare worse with pneumonia. Though pneumonia is the number-one cause of hospitalization in children in the U.S., older adults hospitalized with the disease have a greater risk of death than those hospitalized for any of the other top-10 reasons. That’s why it’s particularly important for older adults to get their RSV, flu and COVID vaccines, Thyagarajan says. “The populations who are at higher risk for complications, hospitalizations and dying from respiratory viruses and bacteria are the same populations who will benefit most from these vaccinations,” she says.
How is pneumonia treated?
Most viral pneumonia can only be treated with supportive care, such as providing oxygen; people with severe cases may require ventilators, heart-lung machines and other forms of mechanical ventilation, Offit says. Bacterial pneumonia is treated with antibiotics.
If you are otherwise healthy, there’s no need to contact a health care provider in the first several days of developing a respiratory infection, Thyagarajan says. But if you develop warning symptoms, such as confusion, shortness of breath or a fever that lasts more than three or four days, “it’s prudent to call your health care provider or seek emergency care,” she says.
Antivirals for flu and COVID , such as Paxlovid, can reduce the likelihood of developing pneumonia when taken early in the course of illness. Those in high-risk groups who develop respiratory symptoms, including those who have a chronic illness or are immunocompromised, should call their health care provider even when the symptoms seem mild, Schaffner says. That way they can get tested for flu and COVID to see if they potentially qualify for medications that reduce the severity of those diseases. Diagnosing an infection and treating it early are key to stopping it from turning into pneumonia.
How can you prevent pneumonia?
Though vaccination can’t prevent all cases of pneumonia, five vaccines recommended in the U.S. can substantially reduce risk of it. Two of these are already routinely recommended for children: the pneumococcal conjugate vaccines (PCV15 and PCV20) and the Haemophilus influenzae (Hib) vaccine . Pneumococcal vaccines are also recommended in adults aged 65 and older, as well as adults with certain medical conditions.
The COVID and seasonal flu vaccines , recommended for everyone aged six months and older, greatly reduce the risk of those diseases developing into pneumonia. Protection against RSV by the monoclonal antibody nirsevimab (Beyfortus) and the recently approved RSV vaccines can also reduce pneumonia risk in those eligible, including adults aged 60 and older, pregnant people, babies and some toddlers.
(Pneumonia develops in one out of five cases of pertussis, or whooping cough, so pertussis vaccination can also prevent pneumonia.)
The same behaviors recommended to prevent the spread of COVID, such as masking, staying home when sick and social distancing, will also reduce risk of other respiratory illnesses that can cause pneumonia.
“If you’re in a high-risk group—you’re older, you’re frail, you have underlying illnesses, you’re immune-compromised—you can get out your mask, and you can be more cautious when you travel or go to the supermarket or any indoor gathering of people,” Schaffner says.
Thyagarajan says she wears her mask at large gatherings in the winter season to protect herself and to protect others as well. That’s especially important if you are a caregiver for an older person or young baby, she adds.
Avoiding people who are coughing and showing other symptoms is obviously ideal, too, but it can be difficult to do, Schaffner adds. Stay home if you are sick—it’s ultimately one of the best ways to avoid spreading illness.
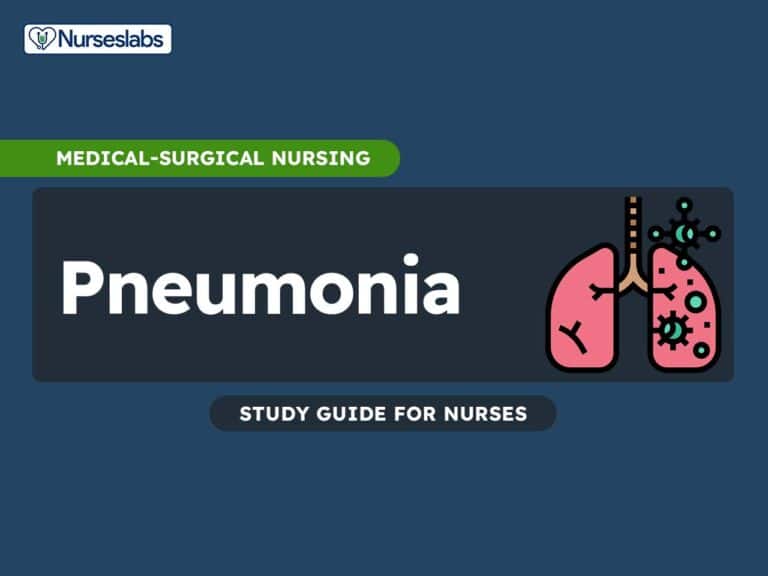
Learn about the nursing care management of patients with pneumonia .
Table of Contents
- What is Pneumonia?
Community-Acquired Pneumonia
Hospital-Acquired Pneumonia
Pneumonia in the Immunocompromised Host
Aspiration pneumonia, pathophysiology, epidemiology.
- Clinical Manifestations
Complications
Assessment and diagnostic findings, medical management, nursing assessment, nursing care planning & goals, nursing priorities, nursing interventions, discharge and home care guidelines, documentation guidelines, what is pneumonia.
Respiratory diseases are rampant today because it is easier spread in crowded areas. Pneumonia is one of the most common respiratory problems and it affects all stages of life.
- Pneumonia is an inflammation of the lung parenchyma caused by various microorganisms, including bacteria, mycobacteria, fungi , and viruses.
- Pneumonitis is a more general term that describes the inflammatory process in the lung tissue that may predispose and place the patient at risk for microbial invasion.
Classification
Pneumonia is classified into four: community-acquired pneumonia (CAP) and hospital-acquired pneumonia (HAP), pneumonia in the immunocompromised host, and aspiration pneumonia.
- CAP occurs either in the community setting or within the first 48 hours after hospitalization .
- The causative agents for CAP that needs hospitalization include streptococcus pneumoniae , H. influenza , Legionella , and Pseudomonas aeruginosa .
- Only in 50% of the cases does the specific etiologic agent become identified.
- Streptococcus pneumoniae is the most common cause of CAP in people younger than 60 years of age.
- Viruses are the most common cause of pneumonia in infants and children.
- HAP is also called nosocomial pneumonia and is defined as the onset of pneumonia symptoms more than 48 hours after admission in patients with no evidence of infection at the time of admission.
- HAP is the most lethal nosocomial infection and the leading cause of death in patients with such infections.
- Common microorganisms that are responsible for HAP include Enterobacter species , Escherichia coli , influenza , Klebsiella species , Proteus , Serratia marcescens , S. aureus , and S. pneumonia .
- The usual presentation of HAP is a new pulmonary infiltrate on chest x-ray combined with evidence of infection.
- Pneumonia in immunocompromised hosts includes Pneumocystis pneumonia, fungal pneumonias and Mycobacterium tuberculosis .
- Patients who are immunocompromised commonly develop pneumonia from organisms of low virulence .
- Pneumonia in immunocompromised hosts may be caused by the organisms also observe in HAP and CAP.
- Aspiration pneumonia refers to the pulmonary consequences resulting from entry of endogenous or exogenous substances into the lower airway.
- The most common form of aspiration pneumonia is a bacterial infection from aspiration of bacteria that normally reside in the upper airways.
- Aspiration pneumonia may occur in the community or hospital setting.
- Common pathogens are S. pneumonia , H.influenza , and S. aureus .
Having an idea about the disease process helps the patient understand the treatment regimen and its importance, increasing patient compliance .
- Pneumonia arises from normal flora present in patients whose resistance has been altered or from aspiration of flora present in the oropharynx.
- An inflammatory reaction may occur in the alveoli, producing exudates that interfere with the diffusion of oxygen and carbon dioxide.
- White blood cells also migrate into the alveoli and fill the normally air-filled spaces .
- Due to secretions and mucosal edema, there are areas of the lung that are not adequately ventilated and cause partial occlusion of the alveoli or bronchi .
- Hypoventilation may follow, causing ventilation-perfusion mismatch.
- Venous blood entering the pulmonary circulation passes through the under ventilated areas and travels to the left side of the heart deoxygenated.
- The mixing of oxygenated and poorly oxygenated blood can result to arterial hypoxemia .
Pneumonia has affected a lot of people, especially those who have a weak immune system. Learning statistics on pneumonia could give you an idea about how many has fallen victim to this respiratory disease.
- Pneumonia and influenza account for nearly 60,000 deaths annually .
- Pneumonia also ranks as the eighth leading cause of death in the United States.
- It is estimated that more than 915, 000 episodes of CAP occur in adults 65 years old and above in the United States.
- HAP accounts for 15% of hospital-acquired infections and is the leading cause of death in patients with such infections.
- The estimated incidence of HAP 4 to 7 episodes per 1000 hospitalizations.
Each type of pneumonia is caused by different and several factors.
- Streptococcus pneumoniae . This is the leading cause of CAP in people younger than 60 years of age without comorbidity and in those 60 years and older with comorbidity.
- H aemophilus influenzae. This causes a type of CAP that frequently affects elderly people and those with comorbid illnesses.
- Mycoplasma pneumoniae.
- Staphylococcus aureus . Staphylococcus pneumonia occurs through inhalation of the organism.
- Impaired host defenses. When the defenses of the body are down, several pathogens may invade the body.
- Comorbid conditions. There are several conditions that lower the immune system, causing bacteria to pool in the lungs and eventually result in pneumonia.
- Supine positioning. When the patient stays in a prolonged supine position, fluid in the lungs pools down and stays stagnant, making it a breeding place for bacteria.
- Prolonged hospitalization. The risk for hospital infections or nosocomial infections increases the longer the patient stays in the hospital.
Clinical Manifestations
Pneumonia varies in its signs and symptoms depending on its type but it is not impossible to diagnose a specific pneumonia through its clinical manifestations.
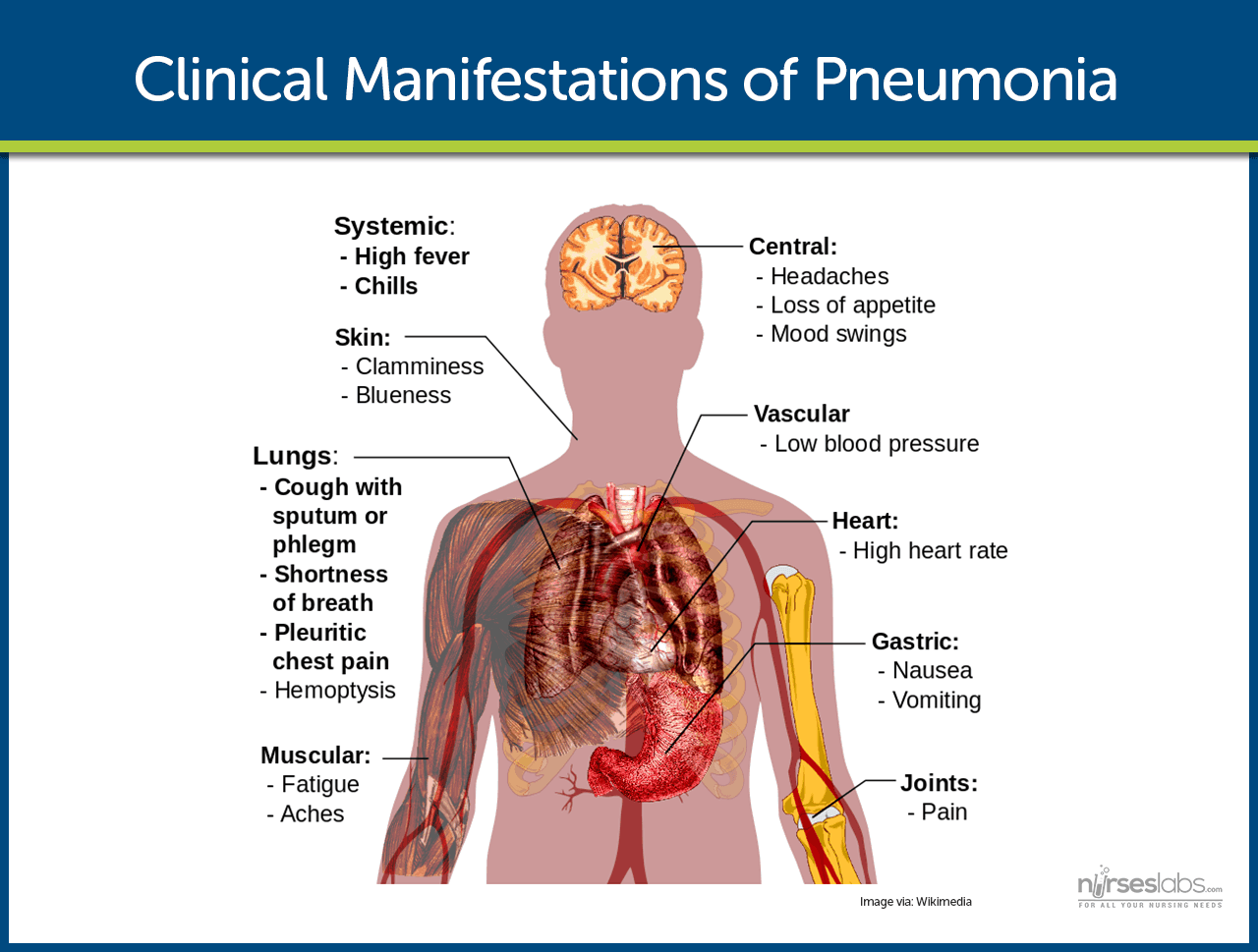
- Rapidly rising fever . Since there is inflammation of the lung parenchyma, fever develops as part of the signs of an infection.
- Pleuritic chest pain . Deep breathing and coughing aggravate the pain in the chest.
- Rapid and bounding pulse. A rapid heartbeat occurs because the body compensates for the low concentration of oxygen in the body.
- Tachypnea. There is fast breathing because the body tries to compensate for the low oxygen concentration in the body.
- Purulent sputum. The sputum becomes purulent because of the infection in the lung parenchyma which produced sputum-filled with pus.
It is better to prevent the occurrence of pneumonia instead of treating the disease itself. Here are several ways that can help prevent pneumonia.
- Pneumococcal vaccine. This vaccine can prevent pneumonia in healthy patients with an efficiency of 65% to 85%.
- Staff education. To help prevent HAP, the CDC (2004) encouraged staff education and involvement in infection prevention.
- Infection and microbiologic surveillance. It is important to carefully observe the infection so that there could be an appropriate application of prevention techniques.
- Modifying host risk for infection . The infection should never be allowed to descend on any host, so the risk must be decreased before it can affect one.
Pneumonia has several complications if left untreated or the interventions are inappropriate. These are the following complications that may develop in patients with pneumonia.
- Shock and respiratory failure. These complications are encountered chiefly in patients who have received no specific treatment and inadequate or delayed treatment.
- Pleural effusion. In pleural effusion , the fluid is sent to the laboratory for analysis, and there are three stages: uncomplicated, complicated, and thoracic empyema.
Assessment and diagnosis of pneumonia must be accurate since there are a lot of respiratory problems that have similar manifestations. The following are assessments and diagnostic tests that could determine pneumonia.
- History taking . The diagnosis of pneumonia is made through history taking, particularly a recent respiratory tract infection.
- Physical examination. Mainly, the number of breaths per minute and breath sounds is assessed during physical examination.
- Chest x-ray. Identifies structural distribution (e.g., lobar, bronchial); may also reveal multiple abscesses/infiltrates, empyema (staphylococcus); scattered or localized infiltration (bacterial); or diffuse/extensive nodular infiltrates (more often viral). In mycoplasmal pneumonia, chest x-ray may be clear.
- Fiberoptic bronchoscopy . May be both diagnostic (qualitative cultures) and therapeutic (re-expansion of lung segment).
- ABGs /pulse oximetry. Abnormalities may be present, depending on extent of lung involvement and underlying lung disease.
- Gram stain/cultures. Sputum collection; needle aspiration of empyema, pleural, and transtracheal or transthoracic fluids; lung biopsies and blood cultures may be done to recover causative organism. More than one type of organism may be present; common bacteria include Diplococcus pneumoniae, Staphylococcus aureus, a-hemolytic streptococcus, Haemophilus influenzae; cytomegalovirus (CMV). Note: Sputum cultures may not identify all offending organisms. Blood cultures may show transient bacteremia.
- CBC. Leukocytosis usually present, although a low white blood cell (WBC) count may be present in viral infection, immunosuppressed conditions such as AIDS , and overwhelming bacterial pneumonia. Erythrocyte sedimentation rate (ESR) is elevated.
- Serologic studies, e.g., viral or Legionella titers, cold agglutinins. Assist in differential diagnosis of specific organism.
- Pulmonary function studies. Volumes may be decreased ( congestion and alveolar collapse); airway pressure may be increased and compliance decreased. Shunting is present (hypoxemia).
- Electrolytes . Sodium and chloride levels may be low.
- Bilirubin. May be increased.
- Percutaneous aspiration/open biopsy of lung tissues. May reveal typical intranuclear and cytoplasmic inclusions (CMV), characteristic giant cells ( rubeola ).
The management of pneumonia centers is a step-by-step process that zeroes on the treatment of the infection through identification of the causative agent.
- Blood culture . Blood culture is performed for identification of the causal pathogen and prompt administration of antibiotics in patients in whom CAP is strongly suspected.
- Administration of macrolides . Macrolides are recommended for people with drug-resistant S. pneumoniae .
- Hydration is an important part of the regimen because fever and tachypnea may result in insensible fluid losses.
- Administration of antipyretics . Antipyretics are used to treat fever and headache.
- Administration of antitussives . Antitussives are used for treatment of the associated cough .
- Bed rest. Complete rest is prescribed until signs of infection are diminished.
- Oxygen administration. Oxygen can be given if hypoxemia develops.
- Pulse oximetry. Pulse oximetry is used to determine the need for oxygen and to evaluate the effectiveness of the therapy.
- Aggressive respiratory measures. Other measures include administration of high concentrations of oxygen, endotracheal intubation, and mechanical ventilation .
Nursing Management
Nurses are expected to perform both dependent and independent functions for the patient to aid him or her towards the restoration of their well-being.
SEE ALSO: 11 Pneumonia Nursing Care Plans for a comprehensive nursing care plan and management guide
Nursing assessment is critical in detecting pneumonia. Here are some tips for your nursing assessment for pneumonia.
- Assess respiratory symptoms. Symptoms of fever, chills, or night sweats in a patient should be reported immediately to the nurse as these can be signs of bacterial pneumonia.
- Assess clinical manifestations. Respiratory assessment should further identify clinical manifestations such as pleuritic pain, bradycardia, tachypnea, and fatigue , use of accessory muscles for breathing, coughing, and purulent sputum.
- Physical assessment. Assess the changes in temperature and pulse; amount, odor, and color of secretions; frequency and severity of cough; degree of tachypnea or shortness of breath; and changes in the chest x-ray findings.
- Assessment in elderly patients. Assess elderly patients for altered mental status , dehydration , unusual behavior, excessive fatigue, and concomitant heart failure .
Through the data collected during assessment, the following nursing diagnoses are made:
- Ineffective airway clearance related to copious tracheobronchial secretions.
- Activity intolerance related to impaired respiratory function.
- Risk for deficient fluid volume related to fever and a rapid respiratory rate.
Planning is essential to establish the interventions that are appropriate for the patient’s condition.
- Improve airway patency.
- Rest to conserve energy.
- Maintenance of proper fluid volume.
- Maintenance of adequate nutrition .
- Understanding of treatment protocol and preventive measures.
- Absence of complications.
- Maintain/improve respiratory function.
- Prevent complications.
- Support recuperative process.
- Provide information about disease process, prognosis, and treatment.
These nursing interventions, if implemented appropriately, would result in the achievement of the goals of the management of pneumonia.
To improve airway patency:
- Removal of secretions. Secretions should be removed because retained secretions interfere with gas exchange and may slow recovery.
- Adequate hydration of 2 to 3 liters per day thins and loosens pulmonary secretions.
- Humidification may loosen secretions and improve ventilation.
- Coughing exercises. An effective, directed cough can also improve airway patency.
- Chest physiotherapy. Chest physiotherapy is important because it loosens and mobilizes secretions.
To promote rest and conserve energy:
- Encourage avoidance of overexertion and possible exacerbation of symptoms.
- Semi-Fowler’s position. The patient should assume a comfortable position to promote rest and breathing and should change positions frequently to enhance secretion clearance and pulmonary ventilation and perfusion.
To promote fluid intake:
- Fluid intake. Increase in fluid intake to at least 2L per day to replace insensible fluid losses.
To maintain nutrition:
- Fluids with electrolytes. This may help provide fluid, calories, and electrolytes.
- Nutrition-enriched beverages. Nutritionally enhanced drinks and shakes can also help restore proper nutrition.
To promote patient’s knowledge:
- Instruct patient and family about the cause of pneumonia, management of symptoms, signs, and symptoms, and the need for follow-up.
- Instruct patient about the factors that may have contributed to the development of the disease.
Expected patient outcomes include the following:
- Demonstrates improved airway patency.
- Rests and conserves energy by limiting activities and remaining in bed while symptomatic and then slowly increasing activities.
- Maintains adequate hydration.
- Consumes adequate dietary intake.
- States explanation for management strategies.
- Complies with management strategies.
- Exhibits no complications.
- Complies with treatment protocol and prevention strategies.
Patient education is crucial regardless of the setting because self-care is essential in achieving a patient’s well-being.
- Oral antibiotics. Teach the patient about the proper administration, potential side effects, and symptoms to report.
- Breathing exercises. Teach the patient breathing exercises to promote secretion clearance and volume expansion.
- Follow-up check up. Strict compliance to follow-up checkups is important to check the latest chest x-ray result or physical examination findings.
- Smoking cessation. Smoking should be stopped because it inhibits tracheobronchial ciliary action and irritates the mucous cells of the bronchi.
Documentation of data must be accurate and up-to-date to avoid unnecessary legal situations that might occur.
- Document breath sounds, presence and character of secretions, use of accessory muscles for breathing.
- Document character of cough and sputum.
- Document respiratory rate, pulse oximetry/O2 saturation, and vital signs.
- Document plan of care and who is involved in planning.
- Document client’s response to interventions, teaching, and actions performed.
- Document if there is use of respiratory devices or airway adjuncts.
- Document response to medications administered.
- Document modifications to plan of care.
See also: Respiratory System NCLEX Practice Questions and Reviewer (220 Questions)
11 thoughts on “Pneumonia”
This is very helpful. Thank you very much. I learned a lot for my upcoming exam next week. May God bless you.
Very useful keep on updating us with such strong information ,may you be blessed 🙏
Wow thanks so much love the website
Hi Marianne, The article is super good. I really appreciate it. Thanks a lot!!!
Thank you I like the explanation and am glad to you
very much thanks to nurseslabs.com
Thanks very much for Nurseslabs
You’re very welcome for the Nurseslabs resources! I’m thrilled to hear that you found the information on pneumonia helpful. Can you share any personal tips or experiences in managing pneumonia cases that have helped you in your nursing studies or clinical practice? Sharing practical insights can be incredibly beneficial for fellow students and aspiring nurses. 🩺💬📚
This is very helpful.Thank you so much.
You wrote : Pneumonia is the most common cause of CAP Did you mean the other way around? thanks
Hi, thanks, this has been updated.
Leave a Comment Cancel reply
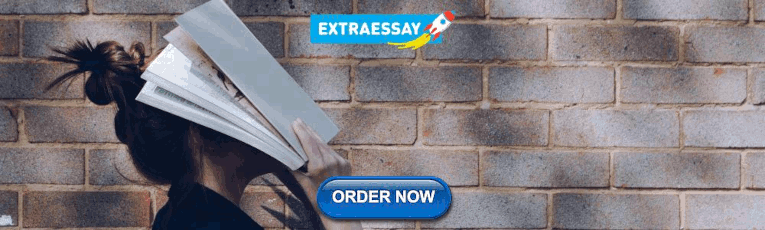
IMAGES
VIDEO
COMMENTS
An 81-year-old man presented with fever, cough, and shortness of breath. Within a few hours after presentation, chest pain and respiratory distress developed. A chest radiograph showed bilateral pa...
Case Presentation. History of Present Illness: A 33-year-old white female presents after admission to the general medical/surgical hospital ward with a chief complaint of shortness of breath on exertion. She reports that she was seen for similar symptoms previously at her primary care physician's office six months ago.
Case Presentation. The patient is a 60-year-old white female presenting to the emergency department with acute onset shortness of breath. Symptoms began approximately 2 days before and had progressively worsened with no associated, aggravating, or relieving factors noted. She had similar symptoms approximately 1 year ago with an acute, chronic ...
Pneumonia (from the Greek pneuma, "breath") is a potentially fatal infection and inflammation of the lower. respiratory tract (i.e., bronchioles and alveoli) usually caused by inhaled bacteria ...
This case report describes common historical and physical examination findings in CABP and the use of traditional and more modern diagnostic tools, as well as treatment dilemmas currently facing clinicians. Streptococcus pneumoniae remains the leading bacterial cause of pneumonia in the United States and globally.
lung, pneumonia, pneumonia, recurrent, pleural effusion, atelectasis A previously healthy 15-year-old girl presents with a history of back pain, chills, and shortness of breath of 1 day's duration. On examination she is afebrile and well appearing despite mild tachypnea (respiratory rate of 24 breaths/min).
The World Health Organization's (WHO) case definition for childhood pneumonia, composed of simple clinical signs of cough, difficult breathing and fast breathing, is widely used in resource poor settings to guide management of acute respiratory infections. The definition is also commonly used as an entry criteria or endpoint in different intervention and disease burden studies.
A US study on the incidence, outcomes and disease burden in >18,000 hospitalized patients with COPD 37 found that, during the 2-year study, 3,419 patients had pneumonia; the annual incidence for ...
In the United States, community-acquired pneumonia is one of the leading causes of hospitalization and death, with approximately 6 million cases reported each year. 1-6 The annual incidence of ...
The present study reports on 3 well-documented cases of CAP due to Corynebacteria (2 cases of C. propinquum, 1 case of C. striatum) that were diagnosed in a 6-month period at a large tertiary care hospital.The study reviews the medical literature on Corynebacterium spp. as etiologic agents of pneumonia, with the goal of increasing awareness of the possibility that so-called "normal ...
This case study of severe Covid-19 pneumonia and CRS illustrates some of the diagnostic and therapeutic challenges and controversies regarding the management of this novel and complex infection. Meticulous monitoring for and early treatment of the hyperinflammatory phase of the disease may be crucial in preventing progression to severe ARDS ...
Background Neurologic impediments occur in only 0.1% of Mycoplasma pneumoniae infections. Although direct intracerebral infection can occur in these patients, autoimmune-mediated reactions secondary to molecular mimicry are the most common pathophysiology of such neurological complications. These complications include immune-mediated encephalitis, peripheral neuritis such as Guillain-Barré ...
Patient was managed with co-trimoxazole 48 hourly and prednisolone 25 mg BID.Case 2: A 70-year old male was admitted with high grade fever and SOB for 4 days. He was a known hypertensive, with a history of severe COVID-19 pneumonia 1.5 months back for which he was managed with IV tocilizumab and dexamethasone. His SpO2 was 63% and RR was 73 BPM.
Read chapter 5 of Infectious Diseases: A Case Study Approach online now, exclusively on AccessPharmacy. AccessPharmacy is a subscription-based resource from McGraw Hill that features trusted pharmacy content from the best minds in the field. ... "Community-Acquired Pneumonia." Infectious Diseases: A Case Study Approach Cho JC. Cho J.C.(Ed.), Ed ...
"Walking pneumonia" is a lay term often used to describe mild pneumonia cases, particularly those caused by Mycoplasma bacteria. It also has been called atypical pneumonia, Thyagarajan says ...
Abstract. The following fictional case is intended as a learning tool within the Pathology Competencies for Medical Education (PCME), a set of national standards for teaching pathology. These are divided into three basic competencies: Disease Mechanisms and Processes, Organ System Pathology, and Diagnostic Medicine and Therapeutic Pathology.
Pneumonia varies in its signs and symptoms depending on its type but it is not impossible to diagnose a specific pneumonia through its clinical manifestations. Rapidly rising fever. Since there is inflammation of the lung parenchyma, fever develops as part of the signs of an infection. Pleuritic chest pain.
This case study aimed to represent the actual scenario of severe childhood pneumonia case management at community clinic. Considering that circumstances, International Centre for Diarrheal Disease Research, Bangladesh (icddr,b) developed an innovative day care management approach as safe, effective and less expensive alternative to hospital ...