
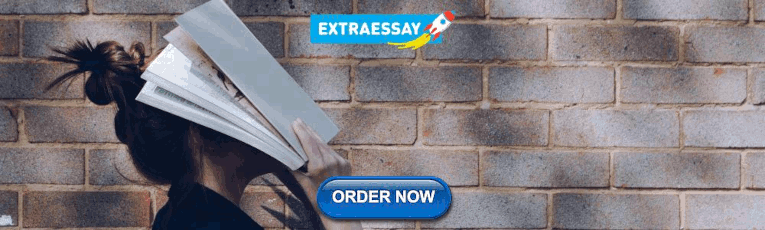
A promising new pathway to treating type 2 diabetes
This year marks the 100th anniversary of the discovery of insulin, a scientific breakthrough that transformed Type 1 diabetes, once known as juvenile diabetes or insulin-dependent diabetes, from a terminal disease into a manageable condition.
Today, Type 2 diabetes is 24 times more prevalent than Type 1. The rise in rates of obesity and incidence of Type 2 diabetes are related and require new approaches, according to University of Arizona researchers, who believe the liver may hold the key to innovative new treatments.
"All current therapeutics for Type 2 diabetes primarily aim to decrease blood glucose. So, they are treating a symptom, much like treating the flu by decreasing the fever," said Benjamin Renquist, an associate professor in the UArizona College of Agriculture and Life Sciences and BIO5 Institute member. "We need another breakthrough."
In two newly published papers in Cell Reports , Renquist, along with researchers from Washington University in St. Louis, the University of Pennsylvania and Northwestern University, outline a new target for Type 2 diabetes treatment.
Renquist, whose research lab aims to address obesity-related diseases, has spent the last nine years working to better understand the correlation between obesity, fatty liver disease and diabetes, particularly how the liver affects insulin sensitivity.
"Obesity is known to be a cause of Type 2 diabetes and, for a long time, we have known that the amount of fat in the liver increases with obesity," Renquist said. "As fat increases in the liver, the incidence of diabetes increases."
This suggested that fat in the liver might be causing Type 2 Diabetes, but how fat in the liver could cause the body to become resistant to insulin or cause the pancreas to over-secrete insulin remained a mystery, Renquist said.
Renquist and his collaborators focused on fatty liver, measuring neurotransmitters released from the liver in animal models of obesity, to better understand how the liver communicates with the brain to influence metabolic changes seen in obesity and diabetes.
"We found that fat in the liver increased the release of the inhibitory neurotransmitter Gamma-aminobutyric acid, or GABA," Renquist said. "We then identified the pathway by which GABA synthesis was occurring and the key enzyme that is responsible for liver GABA production -- GABA transaminase."
A naturally occurring amino acid, GABA is the primary inhibitory neurotransmitter in the central nervous system, meaning it decreases nerve activity.
Nerves provide a conduit by which the brain and the rest of the body communicate. That communication is not only from the brain to other tissues, but also from tissues back to the brain, Renquist explained.
"When the liver produces GABA, it decreases activity of those nerves that run from the liver to the brain. Thus, fatty liver, by producing GABA, is decreasing firing activity to the brain," Renquist said. "That decrease in firing is sensed by the central nervous system, which changes outgoing signals that affect glucose homeostasis."
To determine if increased liver GABA synthesis was causing insulin resistance, graduate students in Renquist's lab, Caroline Geisler and Susma Ghimire, pharmacologically inhibited liver GABA transaminase in animal models of Type 2 diabetes.
"Inhibition of excess liver GABA production restored insulin sensitivity within days," said Geisler, now a postdoctoral researcher at the University of Pennsylvania and lead author on the papers. "Longer term inhibition of GABA-transaminase resulted in decreased food intake and weight loss."
Researchers wanted to ensure the findings would translate to humans. Kendra Miller, a research technician in Renquist's lab, identified variations in the genome near GABA transaminase that were associated with Type 2 diabetes. Collaborating with investigators at Washington University, the researchers showed that in people with insulin resistance, the liver more highly expressed genes involved in GABA production and release.
The findings are the foundation of an Arizona Biomedical Research Commission-funded clinical trial currently underway at Washington University School of Medicine in St. Louis with collaborator Samuel Klein, co-author on the study and a Washington University professor of medicine and nutritional science. The trial will investigate the use of a commercially available Food and Drug Administration-approved inhibitor of GABA transaminase to improve insulin sensitivity in people who are obese.
"A novel pharmacological target is just the first step in application; we are years away from anything reaching the neighborhood pharmacy," Renquist said. "The magnitude of the obesity crisis makes these promising findings an important first step that we hope will eventually impact the health of our family, friends and community."
- Liver Disease
- Chronic Illness
- Diseases and Conditions
- Hormone Disorders
- Diet and Weight Loss
- Personalized Medicine
- Diabetes mellitus type 1
- Diabetes mellitus type 2
- Stem cell treatments
- Liver transplantation
- Sports medicine
Story Source:
Materials provided by University of Arizona . Original written by Rosemary Brandt. Note: Content may be edited for style and length.
Journal Reference :
- Caroline E. Geisler, Susma Ghimire, Stephanie M. Bruggink, Kendra E. Miller, Savanna N. Weninger, Jason M. Kronenfeld, Jun Yoshino, Samuel Klein, Frank A. Duca, Benjamin J. Renquist. A critical role of hepatic GABA in the metabolic dysfunction and hyperphagia of obesity . Cell Reports , 2021; 35 (13): 109301 DOI: 10.1016/j.celrep.2021.109301
Cite This Page :
Explore More
- This Alloy Is Kinky
- Giant Galactic Explosion: Galaxy Pollution
- Flare Erupting Around a Black Hole
- Two Species Interbreeding Created New Butterfly
- Warming Antarctic Deep-Sea and Sea Level Rise
- Octopus Inspires New Suction Mechanism for ...
- Cities Sinking: Urban Populations at Risk
- Puzzle Solved About Ancient Galaxy
- How 3D Printers Can Give Robots a Soft Touch
- Combo of Multiple Health Stressors Harming Bees
Trending Topics
Strange & offbeat.
- Mission & Approach
- The NYSCF Impact
- NYSCF Leadership
- Board of Directors
- Leadership Council
- Junior Leadership Council
- NYSCF Staff
- Partnering & Alliances
- Careers at NYSCF
- NYSCF Summer Internship Program
- Contact NYSCF
- Subscribe to NYSCF Updates
- Research Capabilities
- NYSCF Global Stem Cell Array®
- Repository / Stem Cell Search
Publications
- Alzheimer’s Disease
- Autism Spectrum Disorder
- Macular Degeneration
- Multiple Sclerosis
- Parkinson’s Disease
- Psychiatric Disease
- Women’s Reproductive Cancers
- Opportunities
- Fellowship Awards
- Stem Cell Investigator Awards
- Neuroscience Investigator Awards
- NYSCF – Robertson Stem Cell Prize
- Forms for Grantees
- Students and Teachers
- General Public
- Scientific Community
- Gender Equity in STEM
- Seven Actionable Strategies for Institutions to Improve Gender Equity in STEM
- The Report Card for Gender Equality
- Stem Cells 101
- Glossary & Terms
- Understanding Treatments
- Educational Webinars
- Latest News
- Press Release
- Newsletters
- Upcoming Events
- NYSCF Conference
- NYSCF Gala & Science Fair
- Past Events
- Press & Media Inquiries
- Ways to Give
- Donate Online Now
- Donation FAQs
Diabetes Research at NYSCF
About Diabetes Diabetes News Publications FAQs
At NYSCF, we are dedicated to uncovering diabetes causes and finding a cure for the disease, not just treatments for its symptoms. We are approach this goal in several ways:
- At the NYSCF Research Institute, we are using our own, powerful robotic system for creating stem cells. Our NYSCF Global Stem Cell Array® can rapidly and reproducibly create stem cells from skin or blood, and then reprogram these cells into pancreatic beta cells — the insulin-producing cells affected in diabetes.
- We then study how cells generated from type 1 and type 2 diabetes patients behave, identifying cellular mechanisms that may drive the disease, testing drugs on the cells, and developing strategies to engineer healthy cells for replacement therapies.
- Lastly, we are engineering beta cells that are invisible to the immune system. Type 1 diabetes is an autoimmune disease, and even if we can develop new beta cells to replace the damaged cells, we need a way to make sure the immune system will not attack them. At NYSCF, we are genetically engineering beta cells that can camouflage themselves from the immune system, keeping them safe from destruction.
About Diabetes
Diabetes definition.
Diabetes is a group of diseases characterized by impaired regulation of blood sugar levels by insulin. Insulin, a hormone produced in the pancreas, helps the body extract sugar from our bloodstream and transfer it into our cells, where it is used for energy.
Almost 26 million people have diabetes in the United States alone, and this number continues to grow. There are many different forms of diabetes (including neonatal diabetes and gestational diabetes), but you may have heard of the two major types:
Type 1 diabetes Type 1 diabetics do not produce insulin because their immune system erroneously attacks the cells that produce it.
Type 2 diabetes Type 2 diabetics exhibit insulin resistance or do not produce enough insulin to maintain regular blood sugar levels.
Diabetes Symptoms
Insulin helps the body absorb glucose from the bloodstream, and when it is absent, blood sugar levels can become dangerously high, affecting virtually all tissues in the body and causing health problems such as the following:
- feeling tired all day
- excessive thirst
- blurred vision
- frequent urination
- unexplained weight loss
- increased appetite
- diabetic coma
Diabetes Prevention
Type 1 diabetes is an autoimmune disease that has no reliable prevention strategies at this time. Researchers are currently exploring whether genetics can increase one’s risk for developing type 1 diabetes, but the disease cannot be prevented.
Type 2 diabetes can sometimes be prevented by maintaining a healthy diet, losing weight, and exercising, although genetics play a significant role as well.
Diabetes Treatment
The most common type 1 diabetes treatment is administration of insulin. This can be done through an injection, inhaler, injection port, or through an insulin pump, which delivers insulin continuously throughout the day.
Typical type 2 diabetes treatments and management strategies include weight loss, healthy eating, regular exercise, and in late stages or more severe cases, insulin therapy.
Patients should consult their physician to determine the best treatment course for their symptoms.
Diabetes News
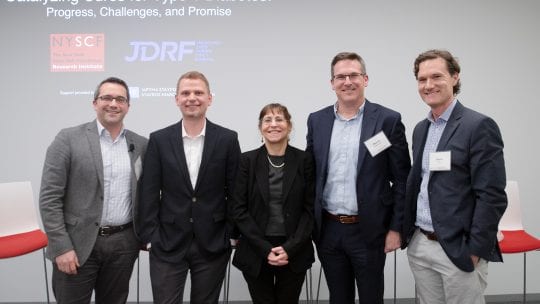
Progress, Challenges, and Promise: On the Road to a Type 1 Diabetes Cure
“How many people were promised a cure [for type 1 diabetes] within five or ten years?” JDRF CEO Aaron...
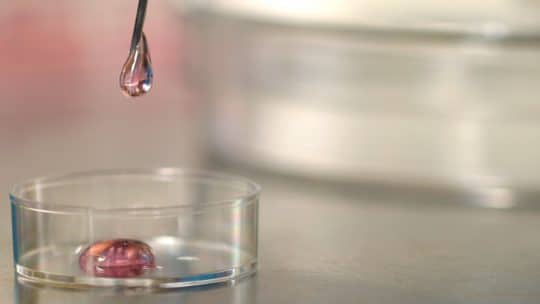
Using Stem Cells and Gene Editing to Fight Neonatal Diabetes
The Context: Neonatal diabetes affects between 1 and 5 percent of all diabetes patients. It is caused by a...
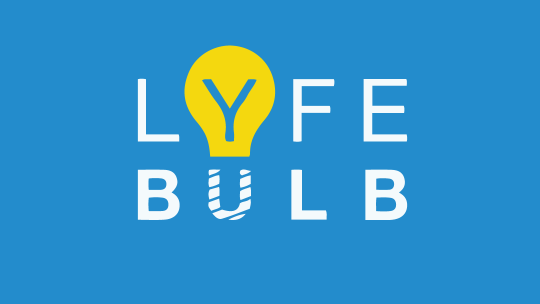
NYSCF and Lyfebulb Announce Collaboration on Chronic Disease Research and Education
Lyfebulb, a patient-empowerment platform that connects chronic disease patients, industry, and investors to empower patients and support user-driven innovation,...
Below are select publications outlining recent advancements in diabetes research from NYSCF scientists.
β-Cell Replacement in Mice Using Human Type 1 Diabetes Nuclear Transfer Embryonic Stem Cells. Sui L, Danzl N, Campbell SR, Viola R, Williams D, Xing Y, Wang Y, Phillips N, Poffenberger G, Johannesson B, Oberholzer J, Powers AC, Leibel RL, Chen X, Sykes M, Egli D. Diabetes . 2018. DOI: 10.2337/db17-0120 This study examines the ability of nuclear transfer embryonic stem cells derived from a patient with type 1 diabetes to differentiate into beta cells.
Toward beta cell replacement for diabetes. Johannesson B, Sui L, Freytes DO, Creusot RJ, Egli D. The EMBO Journal . 2015. DOI: 10.15252/embj.201490685 In this article, NYSCF researchers discuss the promise of cell replacement therapies for treating diabetes.
Human oocytes reprogram adult somatic nuclei to diploid pluripotent stem cells. Yamada M, Johannesson B, Sagi I, Burnett LC, Kort DH, Prosser RW, Paull D, Nestor MW, Freeby M, Greenberg E, Goland RS, Leibel RL, Solomon SL, Benvenisty N, Sauer MV, Egli D. Nature . 2014. DOI: 10.1038/nature13287. This paper outlines how NYSCF scientists created stem cells from diabetic patients through a process called somatic cell nuclear transfer. This was the first time this was achieved using cells from adult individuals.
Beta cell dysfunction due to increased ER stress in a stem cell model of Wolfram syndrome. Linshan Shang, Haiqing Hua, Kylie Foo, Hector Martinez, Kazuhisa Watanabe, Matthew Zimmer, David J Kahler, Matthew Freeby, Wendy Chung, Charles LeDuc, Robin Goland, Rudolph L. Leibel and Dieter Egli. Diabetes. 2014. DOI: 10.2337/db13-0717 In this study, scientists NYSCF and Columbia University Medical Center (CUMC) used stem cells created from the skin of patients with a rare form of diabetes—Wolfram syndrome—to elucidate an important biochemical pathway for beta-cell failure in diabetes.
Diabetes FAQs
According to the Center for Disease Control, a chronic disease is a condition that lasts for 1 year or longer, requires ongoing medical attention, and limits activities of daily living. Diabetes is classified as a chronic disease. Other examples include lupus, heart disease, epilepsy, and arthritis.
Diabetes results from dysfunction in the production and sensing of insulin, a hormone that regulates the amount of glucose (sugar) in the blood. Type 1 diabetes is an autoimmune disease in which the body erroneously attacks insulin. Type 2 diabetes is caused by an inability to produce enough insulin, or the development of insulin resistance.
Insulin helps the body absorb glucose from the bloodstream. When you eat food, sugars from that food are deposited into your bloodstream. Insulin allow for these sugars to leave the bloodstream and enter your cells where they can be used for energy.
Insulin is produced in the pancreas.
Pancreatic beta cells are the cells in the pancreas that produce and release insulin. They are a promising target for drug development and cell replacement therapies, as their depletion is the root of type 1 diabetes.
Blood sugar levels are a measure of the amount of glucose (sugar) in the bloodstream at a certain time. When we eat, sugar from food is deposited into our bloodstream. Insulin then helps regulate the levels of sugar in our blood. If they get too high, one can develop hyperglycemia—a buildup of sugar in the bloodstream that can lead to severe health problems such as diabetic coma and can affect the eyes, kidneys, nerves, and heart. In patients with diabetes, insulin cannot sufficiently regulate blood sugar levels. Diabetics therefore monitor their blood sugar levels through periodic blood glucose testing.
From stem cells to pancreatic β-cells: strategies, applications, and potential treatments for diabetes
- Published: 20 April 2024
Cite this article
- Xingrong Feng 1 ,
- Hongmei Zhang 1 ,
- Shanshan Yang 1 ,
- Daxin Cui 1 ,
- Yanting Wu 1 ,
- Xiaocun Qi 1 &
- Zhiguang Su 1
27 Accesses
Explore all metrics
Loss and functional failure of pancreatic β-cells results in disruption of glucose homeostasis and progression of diabetes. Although whole pancreas or pancreatic islet transplantation serves as a promising approach for β-cell replenishment and diabetes therapy, the severe scarcity of donor islets makes it unattainable for most diabetic patients. Stem cells, particularly induced pluripotent stem cells (iPSCs), are promising for the treatment of diabetes owing to their self-renewal capacity and ability to differentiate into functional β-cells. In this review, we first introduce the development of functional β-cells and their heterogeneity and then turn to highlight recent advances in the generation of β-cells from stem cells and their potential applications in disease modeling, drug discovery and clinical therapy. Finally, we have discussed the current challenges in developing stem cell-based therapeutic strategies for improving the treatment of diabetes. Although some significant technical hurdles remain, stem cells offer great hope for patients with diabetes and will certainly transform future clinical practice.
This is a preview of subscription content, log in via an institution to check access.
Access this article
Price includes VAT (Russian Federation)
Instant access to the full article PDF.
Rent this article via DeepDyve
Institutional subscriptions
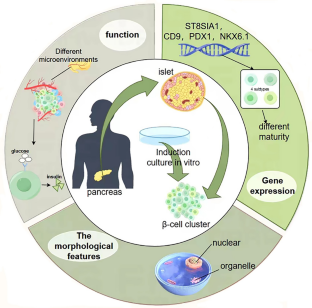
Data availability
No datasets were generated or analysed during the current study.
Sun H, Saeedi P, Karuranga S, Pinkepank M, Ogurtsova K, Duncan BB, Stein C, Basit A, Chan JCN, Mbanya JC, Pavkov ME, Ramachandaran A, Wild SH, James S, Herman WHH, Zhang P, Bommer C, Kuo SH, Boyko EJJ, Magliano DJ (2022) IDF Diabetes Atlas: Global, regional and country-level diabetes prevalence estimates for 2021 and projections for 2045. Diabetes Res Clin Practice. https://doi.org/10.1016/j.diabres.2021.109119
Article Google Scholar
ElSayed NA, Aleppo G, Aroda VR, Bannuru RR, Brown FM, Bruemmer D, Collins BS, Hilliard ME, Isaacs D, Johnson EL, Kahan S, Khunti K, Leon J, Lyons SK, Perry ML, Prahalad P, Pratley RE, Seley JJ, Stanton RC, Gabbay RA, Assoc AD (2023) Classification and diagnosis of diabetes. Diabetes Care 46:S19–S40. https://doi.org/10.2337/dc23-S002
Article CAS PubMed Google Scholar
He SY, Yu XQ, Cui DX, Liu Y, Yang SS, Zhang HM, Hu WX, Su ZG (2023) Nuclear factor-Y mediates pancreatic beta-cell compensation by repressing reactive oxygen species-induced apoptosis under metabolic stress. Chin Med J 136:922–932. https://doi.org/10.1097/Cm9.0000000000002645
Article CAS PubMed PubMed Central Google Scholar
Dal Canto E, Ceriello A, Ryden L, Ferrini M, Hansen TB, Schnell O, Standl E, Beulens JWJ (2019) Diabetes as a cardiovascular risk factor: an overview of global trends of macro and micro vascular complications. Eur J Prev Cardiol 26:25–32. https://doi.org/10.1177/2047487319878371
Zhang XP, Deng D, Cui DX, Liu Y, He SY, Zhang HM, Xie YR, Yu XQ, Yang SS, Chen YL, Su ZG (2022) Cholesterol sulfate exerts protective effect on pancreatic beta-cells by regulating beta-cell mass and insulin secretion. Front Pharmacol. https://doi.org/10.3389/fphar.2022.840406
Article PubMed PubMed Central Google Scholar
Zhang YJ, Guan QY, Liu Y, Zhang YW, Chen YL, Chen JL, Liu YL, Su ZG (2018) Regulation of hepatic gluconeogenesis by nuclear factor Y transcription factor in mice. J Biol Chem 293:7894–7904. https://doi.org/10.1074/jbc.RA117.000508
Ahmad E, Lim S, Lamptey R, Webb DR, Davies MJ (2022) Type 2 diabetes. Lancet 400:1803–1820. https://doi.org/10.1016/S0140-6736(22)01655-5
Article PubMed Google Scholar
Satin LS, Soleimanpour SA, Walker EM (2021) New aspects of diabetes research and therapeutic development. Pharmacol Rev 73:1001–1015. https://doi.org/10.1124/pharmrev.120.000160
Liu Y, He SY, Zhou RX, Zhang XP, Yang SS, Deng D, Zhang CX, Yu XQ, Chen YL, Su ZG (2021) Nuclear factor-Y in mouse pancreatic beta-cells plays a crucial role in glucose homeostasis by regulating beta-cell mass and insulin secretion. Diabetes 70:1703–1716. https://doi.org/10.2337/db20-1238
Rorsman P, Ashcroft FM (2018) Pancreatic beta-cell electrical activity and insulin secretion: of mice and men. Physiol Rev 98:117–214. https://doi.org/10.1152/physrev.00008.2017
Herold KC, Vignali DAA, Cooke A, Bluestone JA (2013) Type 1 diabetes: translating mechanistic observations into effective clinical outcomes. Nat Rev Immunol 13:243–256. https://doi.org/10.1038/nri3422
Cui D, Feng X, Lei S, Zhang H, Hu W, Yang S, Yu X, Su Z (2024) Pancreatic beta-cell failure, clinical implications, and therapeutic strategies in type 2 diabetes. Chin Med J (Engl). https://doi.org/10.1097/CM9.0000000000003034
Zhang X, Yang S, Chen J, Su Z (2018) Unraveling the regulation of hepatic gluconeogenesis. Front Endocrinol (Lausanne) 9:802. https://doi.org/10.3389/fendo.2018.00802
Murphy R, Ellard S, Hattersley AT (2008) Clinical implications of a molecular genetic classification of monogenic beta-cell diabetes. Nat Clin Pract Endocrinol Metab 4:200–213. https://doi.org/10.1038/ncpendmet0778
Basile G, Qadir MMF, Mauvais-Jarvis F, Vetere A, Shoba V, Modell AE, Pastori RL, Russ HA, Wagner BK, Dominguez-Bendala J (2022) Emerging diabetes therapies: bringing back the?-cells. Molecular Metabolism. https://doi.org/10.1016/j.molmet.2022.101477
Gruessner AC, Gruessner RW (2016) Long-term outcome after pancreas transplantation: a registry analysis. Curr Opin Organ Transplant 21:377–385. https://doi.org/10.1097/MOT.0000000000000331
Stratta RJ, Farney AC, Fridell JA (2022) Analyzing outcomes following pancreas transplantation: definition of a failure or failure of a definition. Am J Transplant 22:1523–1526. https://doi.org/10.1111/ajt.17003
Shapiro AM, Lakey JR, Ryan EA, Korbutt GS, Toth E, Warnock GL, Kneteman NM, Rajotte RV (2000) Islet transplantation in seven patients with type 1 diabetes mellitus using a glucocorticoid-free immunosuppressive regimen. N Engl J Med 343:230–238. https://doi.org/10.1056/NEJM200007273430401
Marfil-Garza BA, Shapiro AMJ, Kin T (2021) Clinical islet transplantation: current progress and new frontiers. J Hepatobiliary Pancreat Sci 28:243–254. https://doi.org/10.1002/jhbp.891
Salib A, Cayabyab F, Yoshihara E (2022) Stem cell-derived islets for type 2 diabetes. Int J Mol Sci. https://doi.org/10.3390/ijms23095099
Liu G, David BT, Trawczynski M, Fessler RG (2020) Advances in pluripotent stem cells: history, mechanisms, technologies, and applications. Stem Cell Rev Rep 16:3–32. https://doi.org/10.1007/s12015-019-09935-x
Campbell JE, Newgard CB (2021) Mechanisms controlling pancreatic islet cell function in insulin secretion. Nat Rev Mol Cell Biol 22:142–158. https://doi.org/10.1038/s41580-020-00317-7
Tritschler S, Theis FJ, Lickert H, Böttcher A (2017) Systematic single-cell analysis provides new insights into heterogeneity and plasticity of the pancreas. Mol Metab 6:974–990. https://doi.org/10.1016/j.molmet.2017.06.021
Roscioni SS, Migliorini A, Gegg M, Lickert H (2016) Impact of islet architecture on β-cell heterogeneity, plasticity and function. Nat Rev Endocrinol 12:695–709. https://doi.org/10.1038/nrendo.2016.147
Pan FC, Wright C (2011) Pancreas organogenesis: from bud to plexus to gland. Dev Dyn 240:530–565. https://doi.org/10.1002/dvdy.22584
Shih HP, Wang A, Sander M (2013) Pancreas organogenesis: from lineage determination to morphogenesis. Annu Rev Cell Dev Biol 29:81–105. https://doi.org/10.1146/annurev-cellbio-101512-122405
Collombat P, Mansouri A, Hecksher-Sorensen J, Serup P, Krull J, Gradwohl G, Gruss P (2003) Opposing actions of Arx and Pax4 in endocrine pancreas development. Genes Dev 17:2591–2603. https://doi.org/10.1101/gad.269003
Barsby T, Otonkoski T (2022) Maturation of beta cells: lessons from in vivo and in vitro models. Diabetologia 65:917–930. https://doi.org/10.1007/s00125-022-05672-y
Nishimura W, Iwasa H, Tumurkhuu M (2022) Role of the transcription Factor MAFA in the maintenance of pancreatic β-Cells. Int J Mole Sci. https://doi.org/10.3390/ijms23094478
Jennings RE, Berry AA, Strutt JP, Gerrard DT, Hanley NA (2015) Human pancreas development. Development 142:3126–3137. https://doi.org/10.1242/dev.120063
Nair G, Hebrok M (2015) Islet formation in mice and men: lessons for the generation of functional insulin-producing beta-cells from human pluripotent stem cells. Curr Opin Genet Dev 32:171–180. https://doi.org/10.1016/j.gde.2015.03.004
Russell R, Carnese PP, Hennings TG, Walker EM, Russ HA, Liu JS, Giacometti S, Stein R, Hebrok M (2020) Loss of the transcription factor MAFB limits β-cell derivation from human PSCs. Nat Commun 11:2742. https://doi.org/10.1038/s41467-020-16550-9
Arda HE, Li L, Tsai J, Torre EA, Rosli Y, Peiris H, Spitale RC, Dai C, Gu X, Qu K, Wang P, Wang J, Grompe M, Scharfmann R, Snyder MS, Bottino R, Powers AC, Chang HY, Kim SK (2016) Age-dependent pancreatic gene regulation reveals mechanisms governing human β cell function. Cell Metab 23:909–920. https://doi.org/10.1016/j.cmet.2016.04.002
Cyphert HA, Walker EM, Hang Y, Dhawan S, Haliyur R, Bonatakis L, Avrahami D, Brissova M, Kaestner KH, Bhushan A, Powers AC, Stein R (2019) Examining how the MAFB transcription factor affects islet β-cell function postnatally. Diabetes 68:337–348. https://doi.org/10.2337/db18-0903
Ma Z, Zhang X, Zhong W, Yi H, Chen X, Zhao Y, Ma Y, Song E, Xu T (2023) Deciphering early human pancreas development at the single-cell level. Nat Commun 14:5354. https://doi.org/10.1038/s41467-023-40893-8
Miranda MA, Macias-Velasco JF, Lawson HA (2021) Pancreatic (β-cell heterogeneity in health and diabetes: classes, sources, and subtypes. Am J Physiol-Endocrinol Metab 320:E716–E731. https://doi.org/10.1152/ajpendo.00649.2020
Giordano E, Bosco D, Cirulli V, Meda P (1991) Repeated glucose stimulation reveals distinct and lasting secretion patterns of individual rat pancreatic B cells. J Clin Invest 87:2178–2185. https://doi.org/10.1172/jci115251
Salinno C, Cota P, Bastidas-Ponce A, Tarquis-Medina M, Lickert H, Bakhti M (2019) β-cell maturation and identity in health and disease. Int J Mole Sci. https://doi.org/10.3390/ijms20215417
Dominguez-Gutierrez G, Xin YR, Gromada J (2019) Heterogeneity of human pancreatic beta-cells. Mol Metab 27:S7–S14. https://doi.org/10.1016/j.molmet.2019.06.015
Article CAS PubMed Central Google Scholar
Dorrell C, Schug J, Canaday PS, Russ HA, Tarlow BD, Grompe MT, Horton T, Hebrok M, Streeter PR, Kaestner KH, Grompe M (2016) Human islets contain four distinct subtypes of β cells. Nat Commun 7:11756. https://doi.org/10.1038/ncomms11756
Johnston NR, Mitchell RK, Haythorne E, Pessoa MP, Semplici F, Ferrer J, Piemonti L, Marchetti P, Bugliani M, Bosco D, Berishvili E, Duncanson P, Watkinson M, Broichhagen J, Trauner D, Rutter GA, Hodson DJ (2016) Beta cell hubs dictate pancreatic islet responses to glucose. Cell Metab 24:389–401. https://doi.org/10.1016/j.cmet.2016.06.020
Bader E, Migliorini A, Gegg M, Moruzzi N, Gerdes J, Roscioni SS, Bakhti M, Brandl E, Irmler M, Beckers J, Aichler M, Feuchtinger A, Leitzinger C, Zischka H, Wang-Sattler R, Jastroch M, Tschöp M, Machicao F, Staiger H, Häring HU, Chmelova H, Chouinard JA, Oskolkov N, Korsgren O, Speier S, Lickert H (2016) Identification of proliferative and mature β-cells in the islets of Langerhans. Nature 535:430. https://doi.org/10.1038/nature18624
Li X, Yang KY, Chan VW, Leung KT, Zhang XB, Wong AS, Chong CCN, Wang CC, Ku M, Lui KO (2020) Single-Cell RNA-Seq Reveals that CD9 Is a negative marker of glucose-responsive pancreatic beta-like cells derived from human pluripotent stem cells. Stem Cell Reports 15:1111–1126. https://doi.org/10.1016/j.stemcr.2020.09.009
Dror E, Fagnocchi L, Weqert V, Apostle S, Grimaldi B, Gruber T, Panzeri I, Heyne S, Hoffler KD, Kreiner V, Ching RG, Lu TTH, Semwal A, Johnson B, Senapati P, Lempradl A, Schones D, Imhof A, Shen H, Pospisilik JA (2023) Epigenetic dosage identifies two major and functionally distinct j3 cell subtypes. Cell Metab 35:821. https://doi.org/10.1016/j.cmet.2023.03.008
Aldous N, Moin AM, Abdelalim EM (2023) Pancreatic β-cell heterogeneity in adult human islets and stem cell-derived islets. Cell Mol Life Sci. https://doi.org/10.1007/s00018-023-04815-7
Sarkar A, Saha S, Paul A, Maji A, Roy P, Maity TK (2021) Understanding stem cells and its pivotal role in regenerative medicine. Life Sci. https://doi.org/10.1016/j.lfs.2021.119270
Volarevic V, Markovic BS, Gazdic M, Volarevic A, Jovicic N, Arsenijevic N, Armstrong L, Djonov V, Lako M, Stojkovic M (2018) Ethical and safety issues of stem cell-based therapy. Int J Med Sci 15:36–45. https://doi.org/10.7150/ijms.21666
Keller GM (1995) In-vitro differentiation of embryonic stem-cells. Curr Opin Cell Biol 7:862–869. https://doi.org/10.1016/0955-0674(95)80071-9
Lumelsky N, Blondel O, Laeng P, Velasco I, Ravin R, McKay R (2001) Differentiation of embryonic stem cells to insulin-secreting structures similar to pancreatic islets. Science 292:1389–1394. https://doi.org/10.1126/science.1058866
Rajagopal J, Anderson WJ, Kume S, Martinez OI, Melton DA (2003) Insulin staining of ES cell progeny from insulin uptake. Science 299:363–363
D’Amour KA, Agulnick AD, Eliazer S, Kelly OG, Kroon E, Baetge EE (2005) Efficient differentiation of human embryonic stem cells to definitive endoderm. Nat Biotechnol 23:1534–1541. https://doi.org/10.1038/nbt1163
D’Amour KA, Bang AG, Eliazer S, Kelly OG, Agulnick AD, Smart NG, Moorman MA, Kroon E, Carpenter MK, Baetge EE (2006) Production of pancreatic hormone-expressing endocrine cells from human embryonic stem cells. Nat Biotechnol 24:1392–1401. https://doi.org/10.1038/nbt1259
Jiang JJ, Au M, Lu KH, Eshpeter A, Korbutt G, Fisk G, Majumdar AS (2007) Generation of insulin-producing islet-like clusters from human embryonic stem cells. Stem Cells 25:1940–1953. https://doi.org/10.1634/stemcells.2006-0761
Rezania A, Bruin JE, Arora P, Rubin A, Batushansky I, Asadi A, O’Dwyer S, Quiskamp N, Mojibian M, Albrecht T, Yang YH, Johnson JD, Kieffer TJ (2014) Reversal of diabetes with insulin-producing cells derived in vitro from human pluripotent stem cells. Nat Biotechnol 32:1121–1133. https://doi.org/10.1038/nbt.3033
Pagliuca FW, Millman JR, Gurtler M, Segel M, Van Dervort A, Ryu JH, Peterson QP, Greiner D, Melton DA (2014) Generation of functional human pancreatic beta cells in vitro. Cell 159:428–439. https://doi.org/10.1016/j.cell.2014.09.040
Nair GG, Liu JS, Russ HA, Tran S, Saxton MS, Chen R, Juang C, Li ML, Nguyen VQ, Giacometti S, Puri S, Xing Y, Wang Y, Szot GL, Oberholzer J, Bhushan A, Hebrok M (2019) Recapitulating endocrine cell clustering in culture promotes maturation of human stem-cell-derived beta cells. Nat Cell Biol 21:263–274. https://doi.org/10.1038/s41556-018-0271-4
Golchin A, Chatziparasidou A, Ranjbarvan P, Niknam Z, Ardeshirylajimi A (2021) Embryonic stem cells in clinical trials: current overview of developments and challenges. Adv Exp Med Biol 1312:19–37. https://doi.org/10.1007/5584_2020_592
Maxwell KG, Millman JR (2021) Applications of iPSC-derived beta cells from patients with diabetes. Cell Rep Med 2:100238. https://doi.org/10.1016/j.xcrm.2021.100238
Agrawal A, Narayan G, Gogoi R, Thummer RP (2021) Recent advances in the generation of β-cells from induced pluripotent stem cells as a potential cure for diabetes mellitus. Adv Exp Med Biol 1347:1–27. https://doi.org/10.1007/5584_2021_653
Loretelli C, Assi E, Seelam AJ, Ben Nasr M, Fiorina P (2020) Cell therapy for type 1 diabetes. Expert Opin Biol Ther 20:887–897. https://doi.org/10.1080/14712598.2020.1748596
Veres A, Faust AL, Bushnell HL, Engquist EN, Kenty JHR, Harb G, Poh YC, Sintov E, Gurtler M, Pagliuca FW, Peterson QP, Melton DA (2019) Charting cellular identity during human in vitro beta-cell differentiation. Nature 569:368. https://doi.org/10.1038/s41586-019-1168-5
Augsornworawat P, Maxwell KG, Velazco-Cruz L, Millman JR (2020) Single-Cell Transcriptome Profiling Reveals β Cell Maturation in Stem Cell-Derived Islets after Transplantation. Cell Rep 32:8. https://doi.org/10.1016/j.celrep.2020.108067
Article CAS Google Scholar
Velazco-Cruz L, Song J, Maxwell KG, Goedegebuure MM, Augsornworawat P, Hogrebe NJ, Millman JR (2019) Acquisition of dynamic function in human stem cell-derived beta cells. Stem Cell Reports 12:351–365. https://doi.org/10.1016/j.stemcr.2018.12.012
Hogrebe NJ, Augsornworawat P, Maxwell KG, Velazco-Cruz L, Millman JR (2020) Targeting the cytoskeleton to direct pancreatic differentiation of human pluripotent stem cells. Nat Biotechnol 38:460–470. https://doi.org/10.1038/s41587-020-0430-6
Maxwell KG, Augsornworawat P, Velazco-Cruz L, Kim MH, Asada R, Hogrebe NJ, Morikawa S, Urano F, Millman JR (2020) Gene-edited human stem cell-derived beta cells from a patient with monogenic diabetes reverse preexisting diabetes in mice. Sci Transl Med. https://doi.org/10.1126/scitranslmed.aax9106
Yoshihara E, O’Connor C, Gasser E, Wei Z, Oh TG, Tseng TW, Wang D, Cayabyab F, Dai Y, Yu RT, Liddle C, Atkins AR, Downes M, Evans RM (2020) Immune-evasive human islet-like organoids ameliorate diabetes. Nature 586:606–611. https://doi.org/10.1038/s41586-020-2631-z
Parent AV, Ashe S, Nair GG, Li ML, Chavez J, Liu JS, Zhong YP, Streeter PR, Hebrok M (2022) Development of a scalable method to isolate subsets of stem cell-derived pancreatic islet cells. Stem Cell Reports 17:979–992. https://doi.org/10.1016/j.stemcr.2022.02.001
Sui L, Xin Y, Du Q, Georgieva D, Diedenhofen G, Haataja L, Su Q, Zuccaro MV, Kim J, Fu J, Xing Y, He Y, Baum D, Goland RS, Wang Y, Oberholzer J, Barbetti F, Arvan P, Kleiner S, Egli D (2021) Reduced replication fork speed promotes pancreatic endocrine differentiation and controls graft size. JCI Insight. https://doi.org/10.1172/jci.insight.141553
Shilleh AH, Beard S, Russ HA (2023) Enrichment of stem cell-derived pancreatic beta-like cells and controlled graft size through pharmacological removal of proliferating cells. Stem Cell Reports 18:1284–1294. https://doi.org/10.1016/j.stemcr.2023.05.010
Guan JY, Wang G, Wang JL, Zhang ZY, Fu Y, Cheng L, Meng GF, Lyu YL, Zhu JL, Li YQ, Wang YL, Liuyang SJ, Liu B, Yang ZR, He HJ, Zhong XX, Chen QJ, Zhang X, Sun SC, Lai WF, Shi Y, Liu LL, Wang LP, Li C, Lu SC, Deng HK (2022) Chemical reprogramming of human somatic cells to pluripotent stem cells. Nature 605:325. https://doi.org/10.1038/s41586-022-04593-5
Du YY, Liang Z, Wang S, Sun D, Wang XF, Liew SY, Lu SY, Wu SS, Jiang Y, Wang YQ, Zhang BY, Yu WH, Lu Z, Pu Y, Zhang Y, Long HT, Xiao SS, Liang R, Zhang ZY, Guan JY, Wang JL, Ren HX, Wei YL, Zhao JX, Sun SC, Liu TL, Meng GF, Wang L, Gu JB, Wang T, Liu YN, Li C, Tang C, Shen ZY, Peng XZ, Deng HK (2022) Human pluripotent stem-cell-derived islets ameliorate diabetes in non-human primates. Nat Med 28:2725. https://doi.org/10.1038/s41591-021-01645-7
Liang Z, Sun D, Lu SY, Lei ZJ, Wang SS, Luo ZF, Zhan JQ, Wu SS, Jiang Y, Lu Z, Sun SC, Shi YY, Long HT, Wei YL, Yu WH, Wang ZH, Yi LS, Zhang Y, Sun WY, Fang XF, Li YY, Lu SF, Lv JY, Sui WG, Shen ZY, Peng XZ, Du YY, Deng HK (2023) Implantation underneath the abdominal anterior rectus sheath enables effective and functional engraftment of stem-cell-derived islets. Nat Metab. https://doi.org/10.1038/s42255-022-00713-7
Ghoneim MA, Refaie AF, Elbassiouny BL, Gabr MM, Zakaria MM (2020) From mesenchymal stromal/stem cells to insulin-producing cells: progress and challenges. Stem Cell Rev Rep 16:1156–1172. https://doi.org/10.1007/s12015-020-10036-3
Wu X, Jiang J, Gu Z, Zhang J, Chen Y, Liu X (2020) Mesenchymal stromal cell therapies: immunomodulatory properties and clinical progress. Stem Cell Res Ther 11:345. https://doi.org/10.1186/s13287-020-01855-9
Berman DM, Willman MA, Han D, Kleiner G, Kenyon NM, Cabrera O, Karl JA, Wiseman RW, O’Connor DH, Bartholomew AM, Kenyon NS (2010) Mesenchymal stem cells enhance allogeneic islet engraftment in nonhuman primates. Diabetes 59:2558–2568. https://doi.org/10.2337/db10-0136
Tang YY, Zhou Y, Li HJ (2021) Advances in mesenchymal stem cell exosomes: a review. Stem Cell Res Ther. https://doi.org/10.1186/s13287-021-02138-7
Margiana R, Markov A, Zekiy AO, Hamza MU, Al-Dabbagh KA, Al-Zubaidi SH, Hameed NM, Ahmad I, Sivaraman R, Kzar HH, Al-Gazally ME, Mustafa YF, Siahmansouri H (2022) Clinical application of mesenchymal stem cell in regenerative medicine: a narrative review. Stem Cell Res Ther. https://doi.org/10.1186/s13287-022-03054-0
Kadam S, Muthyala S, Nair P, Bhonde R (2010) Human placenta-derived mesenchymal stem cells and islet-like cell clusters generated from these cells as a novel source for stem cell therapy in diabetes. Rev Diabet Stud 7:168–182. https://doi.org/10.1900/rds.2010.7.168
Zhang Y, Shen W, Hua J, Lei A, Lv C, Wang H, Yang C, Gao Z, Dou Z (2010) Pancreatic islet-like clusters from bone marrow mesenchymal stem cells of human first-trimester abortus can cure streptozocin-induced mouse diabetes. Rejuvenation Res 13:695–706. https://doi.org/10.1089/rej.2009.1016
Path G, Perakakis N, Mantzoros CS, Seufert J (2019) Stem cells in the treatment of diabetes mellitus - Focus on mesenchymal stem cells. Metabolism 90:1–15. https://doi.org/10.1016/j.metabol.2018.10.005
Eydian Z, Mohammad Ghasemi A, Ansari S, Kamali AN, Khosravi M, Momtaz S, Riki S, Rafighdoost L, Entezari Heravi R (2022) Differentiation of multipotent stem cells to insulin-producing cells for treatment of diabetes mellitus: bone marrow- and adipose tissue-derived cells comparison. Mol Biol Rep 49:3539–3548. https://doi.org/10.1007/s11033-022-07194-7
Cho J, D’Antuono M, Glicksman M, Wang J, Jonklaas J (2018) A review of clinical trials: mesenchymal stem cell transplant therapy in type 1 and type 2 diabetes mellitus. Am J Stem Cells 7:82–93
CAS PubMed PubMed Central Google Scholar
Millman JR, Xie C, Van Dervort A, Gürtler M, Pagliuca FW, Melton DA (2016) Generation of stem cell-derived β-cells from patients with type 1 diabetes. Nat Commun 7:11463. https://doi.org/10.1038/ncomms11463
Leite NC, Sintov E, Meissner TB, Brehm MA, Greiner DL, Harlan DM, Melton DA (2020) Modeling type 1 diabetes in vitro using human pluripotent stem cells. Cell Rep 32:107894. https://doi.org/10.1016/j.celrep.2020.107894
Shojima N, Yamauchi T (2023) Progress in genetics of type 2 diabetes and diabetic complications. J Diabetes Investig 14:503–515. https://doi.org/10.1111/jdi.13970
Zeng H, Guo M, Zhou T, Tan L, Chong CN, Zhang T, Dong X, Xiang JZ, Yu AS, Yue L, Qi Q, Evans T, Graumann J, Chen S (2016) An isogenic human ESC platform for functional evaluation of genome-wide-association-study-identified diabetes genes and drug discovery. Cell Stem Cell 19:326–340. https://doi.org/10.1016/j.stem.2016.07.002
Wen XJ, Yang YS (2017) Emerging roles of GLIS3 in neonatal diabetes, type 1 and type 2 diabetes. J Mol Endocrinol 58:R73–R85. https://doi.org/10.1530/Jme-16-0232
Amin S, Cook B, Zhou T, Ghazizadeh Z, Lis R, Zhang T, Khalaj M, Crespo M, Perera M, Xiang JZ, Zhu Z, Tomishima M, Liu C, Naji A, Evans T, Huangfu D, Chen S (2018) Discovery of a drug candidate for GLIS3-associated diabetes. Nat Commun 9:2681. https://doi.org/10.1038/s41467-018-04918-x
Hussain MA, Akalestou E, Song WJ (2016) Inter-organ communication and regulation of beta cell function. Diabetologia 59:659–667. https://doi.org/10.1007/s00125-015-3862-7
Picollet-D’hahan N, Zuchowska A, Lemeunier I, Le Gac S (2021) Multiorgan-on-a-Chip: a systemic approach to model and decipher inter-organ communication. Trends Biotechnol 39:788–810. https://doi.org/10.1016/j.tibtech.2020.11.014
Bauer S, Wennberg Huldt C, Kanebratt KP, Durieux I, Gunne D, Andersson S, Ewart L, Haynes WG, Maschmeyer I, Winter A, Ämmälä C, Marx U, Andersson TB (2017) Functional coupling of human pancreatic islets and liver spheroids on-a-chip: towards a novel human ex vivo type 2 diabetes model. Sci Rep 7:14620. https://doi.org/10.1038/s41598-017-14815-w
Yamashita-Sugahara Y, Matsumoto M, Ohtaka M, Nishimura K, Nakanishi M, Mitani K, Okazaki Y (2016) An inhibitor of fibroblast growth factor receptor-1 (FGFR1) promotes late-stage terminal differentiation from NGN3+ pancreatic endocrine progenitors. Sci Rep 6:35908. https://doi.org/10.1038/srep35908
Kimura A, Toyoda T, Nishi Y, Nasu M, Ohta A, Osafune K (2017) Small molecule AT7867 proliferates PDX1-expressing pancreatic progenitor cells derived from human pluripotent stem cells. Stem Cell Res 24:61–68. https://doi.org/10.1016/j.scr.2017.08.010
Korostylev A, Mahaddalkar PU, Keminer O, Hadian K, Schorpp K, Gribbon P, Lickert H (2017) A high-content small molecule screen identifies novel inducers of definitive endoderm. Mol Metab 6:640–650. https://doi.org/10.1016/j.molmet.2017.04.009
Zhou T, Kim TW, Chong CN, Tan L, Amin S, Badieyan ZS, Mukherjee S, Ghazizadeh Z, Zeng H, Guo M, Crespo M, Zhang T, Kenyon R, Robinson CL, Apostolou E, Wang H, Xiang JZ, Evans T, Studer L, Chen SB (2018) A hPSC-based platform to discover gene-environment interactions that impact human β-cell and dopamine neuron survival. Nat Commun. https://doi.org/10.1038/s41467-018-07201-1
Wei Z, Yoshihara E, He N, Hah N, Fan W, Pinto AFM, Huddy T, Wang Y, Ross B, Estepa G, Dai Y, Ding N, Sherman MH, Fang S, Zhao X, Liddle C, Atkins AR, Yu RT, Downes M, Evans RM (2018) Vitamin D switches BAF complexes to protect β cells. Cell 173:1135-1149.e15. https://doi.org/10.1016/j.cell.2018.04.013
Bashor CJ, Hilton IB, Bandukwala H, Smith DM, Veiseh O (2022) Engineering the next generation of cell-based therapeutics. Nat Rev Drug Discov 21:655–675. https://doi.org/10.1038/s41573-022-00476-6
Wang X, Gao M, Wang Y, Zhang Y (2022) The progress of pluripotent stem cell-derived pancreatic β-cells regeneration for diabetic therapy. Front Endocrinol (Lausanne) 13:927324. https://doi.org/10.3389/fendo.2022.927324
Ramzy A, Thompson DM, Ward-Hartstonge KA, Ivison S, Cook L, Garcia RV, Loyal J, Kim PTW, Warnock GL, Levings MK, Kieffer TJ (2021) Implanted pluripotent stem-cell-derived pancreatic endoderm cells secrete glucose-responsive C-peptide in patients with type 1 diabetes. Cell Stem Cell 28:2047-2061.e5. https://doi.org/10.1016/j.stem.2021.10.003
Shapiro AMJ, Thompson D, Donner TW, Bellin MD, Hsueh W, Pettus J, Wilensky J, Daniels M, Wang RM, Brandon EP, Jaiman MS, Kroon EJ, D’Amour KA, Foyt HL (2021) Insulin expression and C-peptide in type 1 diabetes subjects implanted with stem cell-derived pancreatic endoderm cells in an encapsulation device. Cell Rep Med. https://doi.org/10.1016/j.xcrm.2021.100466
Russ HA, Parent AV, Ringler JJ, Hennings TG, Nair GG, Shveygert M, Guo T, Puri S, Haataja L, Cirulli V, Blelloch R, Szot GL, Arvan P, Hebrok M (2015) Controlled induction of human pancreatic progenitors produces functional beta-like cells in vitro. EMBO J 34:1759–1772. https://doi.org/10.15252/embj.201591058
Agulnick AD, Ambruzs DM, Moorman MA, Bhoumik A, Cesario RM, Payne JK, Kelly JR, Haakmeester C, Srijemac R, Wilson AZ, Kerr J, Frazier MA, Kroon EJ, D’Amour KA (2015) Insulin-producing endocrine cells differentiated in vitro from human embryonic stem cells function in macroencapsulation devices in vivo. Stem Cells Transl Med 4:1214–1222. https://doi.org/10.5966/sctm.2015-0079
Nostro MC, Sarangi F, Yang C, Holland A, Elefanty AG, Stanley EG, Greiner DL, Keller G (2015) Efficient generation of NKX6-1+ pancreatic progenitors from multiple human pluripotent stem cell lines. Stem Cell Rep 4:591–604. https://doi.org/10.1016/j.stemcr.2015.02.017
Memon B, Karam M, Al-Khawaga S, Abdelalim EM (2018) Enhanced differentiation of human pluripotent stem cells into pancreatic progenitors co-expressing PDX1 and NKX6.1. Stem Cell Res Ther 9:15. https://doi.org/10.1186/s13287-017-0759-z
Desai T, Shea LD (2017) Advances in islet encapsulation technologies. Nat Rev Drug Discov 16:338–350. https://doi.org/10.1038/nrd.2016.232
Wu S, Wang L, Fang Y, Huang H, You X, Wu J (2021) Advances in encapsulation and delivery strategies for islet transplantation. Adv Healthc Mater 10:e2100965. https://doi.org/10.1002/adhm.202100965
Vaithilingam V, Bal S, Tuch BE (2017) Encapsulated islet transplantation: where do we stand? Rev Diabet Stud 14:51–78. https://doi.org/10.1900/rds.2017.14.51
Rafael E, Wernerson A, Arner P, Wu GS, Tibell A (1999) In vivo evaluation of glucose permeability of an immunoisolation device intended for islet transplantation: a novel application of the microdialysis technique. Cell Transplant 8:317–326. https://doi.org/10.1177/096368979900800302
Motté E, Szepessy E, Suenens K, Stangé G, Bomans M, Jacobs-Tulleneers-Thevissen D, Ling Z, Kroon E, Pipeleers D (2014) Composition and function of macroencapsulated human embryonic stem cell-derived implants: comparison with clinical human islet cell grafts. Am J Physiol Endocrinol Metab 307:E838–E846. https://doi.org/10.1152/ajpendo.00219.2014
Robert T, De Mesmaeker I, Stangé GM, Suenens KG, Ling Z, Kroon EJ, Pipeleers DG (2018) Functional beta cell mass from device-encapsulated HESC-derived pancreatic endoderm achieving metabolic control. Stem Cell Rep 10:739–750. https://doi.org/10.1016/j.stemcr.2018.01.040
Bruin JE, Rezania A, Xu J, Narayan K, Fox JK, O’Neil JJ, Kieffer TJ (2013) Maturation and function of human embryonic stem cell-derived pancreatic progenitors in macroencapsulation devices following transplant into mice. Diabetologia 56:1987–1998. https://doi.org/10.1007/s00125-013-2955-4
Zhang Q, Gonelle-Gispert C, Li Y, Geng Z, Gerber-Lemaire S, Wang Y, Buhler L (2022) Islet encapsulation: new developments for the treatment of type 1 diabetes. Front Immunol 13:869984. https://doi.org/10.3389/fimmu.2022.869984
Samojlik MM, Stabler CL (2021) Designing biomaterials for the modulation of allogeneic and autoimmune responses to cellular implants in type 1 diabetes. Acta Biomater 133:87–101. https://doi.org/10.1016/j.actbio.2021.05.039
Teramura Y, Iwata H (2009) Islet encapsulation with living cells for improvement of biocompatibility. Biomaterials 30:2270–2275. https://doi.org/10.1016/j.biomaterials.2009.01.036
Cui W, Khan KM, Ma X, Chen G, Desai CS (2020) Human amniotic epithelial cells and human amniotic membrane as a vehicle for islet cell transplantation. Trans Proc 52:982–986. https://doi.org/10.1016/j.transproceed.2020.01.022
Sabek OM, Ferrati S, Fraga DW, Sih J, Zabre EV, Fine DH, Ferrari M, Gaber AO, Grattoni A (2013) Characterization of a nanogland for the autotransplantation of human pancreatic islets. Lab Chip 13:3675–3688. https://doi.org/10.1039/c3lc50601k
Barra JM, Kozlovskaya V, Burnette KS, Banerjee RR, Fraker CA, Kharlampieva E, Tse HM (2023) Localized cytotoxic T cell-associated antigen 4 and antioxidant islet encapsulation alters macrophage signaling and induces regulatory and anergic T cells to enhance allograft survival. Am J Trans 23:498–511. https://doi.org/10.1016/j.ajt.2023.01.007
Elisseeff J, Badylak SF, Boeke JD (2021) Immune and genome engineering as the future of transplantable tissue. N Engl J Med 385:2451–2462. https://doi.org/10.1056/NEJMra1913421
Ghoneim MA, Gabr MM, El-Halawani SM, Refaie AF (2024) Current status of stem cell therapy for type 1 diabetes: a critique and a prospective consideration. Stem Cell Res Ther 15:23. https://doi.org/10.1186/s13287-024-03636-0
Deuse T, Hu X, Gravina A, Wang D, Tediashvili G, De C, Thayer WO, Wahl A, Garcia JV, Reichenspurner H, Davis MM, Lanier LL, Schrepfer S (2019) Hypoimmunogenic derivatives of induced pluripotent stem cells evade immune rejection in fully immunocompetent allogeneic recipients. Nat Biotechnol 37:252–258. https://doi.org/10.1038/s41587-019-0016-3
Han X, Wang M, Duan S, Franco PJ, Kenty JH, Hedrick P, Xia Y, Allen A, Ferreira LMR, Strominger JL, Melton DA, Meissner TB, Cowan CA (2019) Generation of hypoimmunogenic human pluripotent stem cells. Proc Natl Acad Sci U S A 116:10441–10446. https://doi.org/10.1073/pnas.1902566116
Gerace D, Zhou Q, Kenty JH, Veres A, Sintov E, Wang X, Boulanger KR, Li H, Melton DA (2023) Engineering human stem cell-derived islets to evade immune rejection and promote localized immune tolerance. Cell Rep Med 4:100879. https://doi.org/10.1016/j.xcrm.2022.100879
Santini-Gonzalez J, Castro-Gutierrez R, Becker MW, Rancourt C, Russ HA, Phelps EA (2022) Human stem cell derived beta-like cells engineered to present PD-L1 improve transplant survival in NOD mice carrying human HLA class I. Front Endocrinol (Lausanne) 13:989815. https://doi.org/10.3389/fendo.2022.989815
Hu X, Gattis C, Olroyd AG, Friera AM, White K, Young C, Basco R, Lamba M, Wells F, Ankala R, Dowdle WE, Lin A, Egenberger K, Rukstalis JM, Millman JR, Connolly AJ, Deuse T, Schrepfer S (2023) Human hypoimmune primary pancreatic islets avoid rejection and autoimmunity and alleviate diabetes in allogeneic humanized mice. Sci Transl Med 15:eadg5794. https://doi.org/10.1126/scitranslmed.adg5794
Hu X, White K, Olroyd AG, DeJesus R, Dominguez AA, Dowdle WE, Friera AM, Young C, Wells F, Chu EY, Ito CE, Krishnapura H, Jain S, Ankala R, McGill TJ, Lin A, Egenberger K, Gagnon A, Michael Rukstalis J, Hogrebe NJ, Gattis C, Basco R, Millman JR, Kievit P, Davis MM, Lanier LL, Connolly AJ, Deuse T, Schrepfer S (2024) Hypoimmune induced pluripotent stem cells survive long term in fully immunocompetent, allogeneic rhesus macaques. Nat Biotechnol 42:413–423. https://doi.org/10.1038/s41587-023-01784-x
Bergström M, Yao M, Müller M, Korsgren O, von Zur-Mühlen B, Lundgren T (2021) Autologous regulatory T cells in clinical intraportal allogenic pancreatic islet transplantation. Transpl Int 34:2816–2823. https://doi.org/10.1111/tri.14163
Herold KC, Bundy BN, Long SA, Bluestone JA, DiMeglio LA, Dufort MJ, Gitelman SE, Gottlieb PA, Krischer JP, Linsley PS, Marks JB, Moore W, Moran A, Rodriguez H, Russell WE, Schatz D, Skyler JS, Tsalikian E, Wherrett DK, Ziegler AG, Greenbaum CJ (2019) An anti-CD3 antibody, Teplizumab, in relatives at risk for type 1 diabetes. N Engl J Med 381:603–613. https://doi.org/10.1056/NEJMoa1902226
Merani S, Toso C, Emamaullee J, Shapiro AM (2008) Optimal implantation site for pancreatic islet transplantation. Br J Surg 95:1449–1461. https://doi.org/10.1002/bjs.6391
Addison P, Fatakhova K, Rodriguez Rilo HL (2020) Considerations for an alternative site of islet cell transplantation. J Diabetes Sci Technol 14:338–344. https://doi.org/10.1177/1932296819868495
Inagaki A, Imura T, Nakamura Y, Ohashi K, Goto M (2021) The liver surface is an attractive transplant site for pancreatic islet transplantation. J Clin Med. https://doi.org/10.3390/jcm10040724
Pepper AR, Gala-Lopez B, Pawlick R, Merani S, Kin T, Shapiro AM (2015) A prevascularized subcutaneous device-less site for islet and cellular transplantation. Nat Biotechnol 33:518–523. https://doi.org/10.1038/nbt.3211
Kinney SM, Ortaleza K, Vlahos AE, Sefton MV (2022) Degradable methacrylic acid-based synthetic hydrogel for subcutaneous islet transplantation. Biomaterials 281:121342. https://doi.org/10.1016/j.biomaterials.2021.121342
Pellicciaro M, Vella I, Lanzoni G, Tisone G, Ricordi C (2017) The greater omentum as a site for pancreatic islet transplantation. CellR4 Repair Replace Regen Reprogram 5:e2410
PubMed PubMed Central Google Scholar
Damyar K, Farahmand V, Whaley D, Alexander M, Lakey JRT (2021) An overview of current advancements in pancreatic islet transplantation into the omentum. Islets 13:115–120. https://doi.org/10.1080/19382014.2021.1954459
Yasunami Y, Lacy PE, Finke EH (1983) A new site for islet transplantation–a peritoneal-omental pouch. Transplantation 36:181–182. https://doi.org/10.1097/00007890-198308000-00014
Ao ZL, Matayoshi K, Lakey JRT, Rajotte RV, Warnock GL (1993) Survival and function of purified islets in the omental pouch site of outbred dogs. Transplantation 56:524–529. https://doi.org/10.1097/00007890-199309000-00007
Deng H, Zhang A, Pang DRR, Xi Y, Yang Z, Matheson R, Li G, Luo H, Lee KM, Fu Q, Zou Z, Chen T, Wang Z, Rosales IA, Peters CW, Yang J, Coronel MM, Yolcu ES, Shirwan H, García AJ, Markmann JF, Lei J (2023) Bioengineered omental transplant site promotes pancreatic islet allografts survival in non-human primates. Cell Rep Med 4:100959. https://doi.org/10.1016/j.xcrm.2023.100959
Schwarzer A, Talbot SR, Selich A, Morgan M, Schott JW, Dittrich-Breiholz O, Bastone AL, Weigel B, Ha TC, Dziadek V, Gijsbers R, Thrasher AJ, Staal FJT, Gaspar HB, Modlich U, Schambach A, Rothe M (2021) Predicting genotoxicity of viral vectors for stem cell gene therapy using gene expression-based machine learning. Mol Ther 29:3383–3397. https://doi.org/10.1016/j.ymthe.2021.06.017
Hentze H, Soong PL, Wang ST, Phillips BW, Putti TC, Dunn NR (2009) Teratoma formation by human embryonic stem cells: Evaluation of essential parameters for future safety studies. Stem Cell Res 2:198–210. https://doi.org/10.1016/j.scr.2009.02.002
Pellegrini S, Zamarian V, Sordi V (2022) Strategies to improve the safety of iPSC-derived β cells for β cell replacement in diabetes. Trans Int. https://doi.org/10.3389/ti.2022.10575
Jiang W, Sui X, Zhang DH, Liu M, Ding MX, Shi Y, Deng HK (2011) CD24: a novel surface marker for PDX1-positive pancreatic progenitors derived from human embryonic stem cells. Stem Cells 29:609–617. https://doi.org/10.1002/stem.608
Ameri J, Borup R, Prawiro C, Ramond C, Schachter KA, Scharfmann R, Semb H (2017) Efficient generation of glucose-responsive beta cells from isolated GP2 human pancreatic progenitors. Cell Rep 19:36–49. https://doi.org/10.1016/j.celrep.2017.03.032
Cogger KF, Sinha A, Sarangi F, McGaugh EC, Saunders D, Dorrell C, Mejia-Guerrero S, Aghazadeh Y, Rourke JL, Screaton RA, Grompe M, Streeter PR, Powers AC, Brissova M, Kislinger T, Nostro MC (2017) Glycoprotein 2 is a specific cell surface marker of human pancreatic progenitors. Nat Commun. https://doi.org/10.1038/s41467-017-00561-0
Mahaddalkar PU, Scheibner K, Pfluger S, Ansarullah SM, Beckenbauer J, Irmler M, Beckers J, Knöbel S, Lickert H (2020) Generation of pancreatic β cells from CD177 anterior definitive endoderm. Nat Biotechnol 38:1061. https://doi.org/10.1038/s41587-020-0492-5
Salinno C, Büttner M, Cota P, Tritschler S, Tarquis-Medina M, Bastidas-Ponce A, Scheibner K, Burtscher I, Böttcher A, Theis FJ, Bakhti M, Lickert H (2021) CD81 marks immature and dedifferentiated pancreatic β-cells. Mol Metab. https://doi.org/10.1016/j.molmet.2021.101188
Tang C, Lee AS, Volkmer JP, Sahoo D, Nag D, Mosley AR, Inlay MA, Ardehali R, Chavez SL, Pera RR, Behr B, Wu JC, Weissman IL, Drukker M (2011) An antibody against SSEA-5 glycan on human pluripotent stem cells enables removal of teratoma-forming cells. Nat Biotechnol 29:829-U86. https://doi.org/10.1038/nbt.1947
Pellegrini S, Zamarian V, Sordi V (2022) Strategies to improve the safety of iPSC-derived beta cells for beta cell replacement in diabetes. Transpl Int 35:10575. https://doi.org/10.3389/ti.2022.10575
Sheikh S, Ernst D, Keating A (2021) Prodrugs and prodrug-activated systems in gene therapy. Mol Ther 29:1716–1728. https://doi.org/10.1016/j.ymthe.2021.04.006
Nagashima T, Shimizu K, Matsumoto R, Honda H (2018) Selective elimination of human induced pluripotent stem cells using medium with high concentration of L-alanine. Sci Rep 8:12427. https://doi.org/10.1038/s41598-018-30936-2
Qadir MMF, Alvarez-Cubela S, Belle K, Sapir T, Messaggio F, Johnson KB, Umland O, Hardin D, Klein D, Perez-Alvarez I, Sadiq F, Alcazar O, Inverardi LA, Ricordi C, Buchwald P, Fraker CA, Pastori RL, Dominguez-Bendala J (2019) A double fail-safe approach to prevent tumorigenesis and select pancreatic beta cells from human embryonic stem cells. Stem Cell Reports 12:611–623. https://doi.org/10.1016/j.stemcr.2019.01.012
Di Stasi A, Tey SK, Dotti G, Fujita Y, Kennedy-Nasser A, Martinez C, Straathof K, Liu E, Durett AG, Grilley B, Liu H, Cruz CR, Savoldo B, Gee AP, Schindler J, Krance RA, Heslop HE, Spencer DM, Rooney CM, Brenner MK (2011) Inducible apoptosis as a safety switch for adoptive cell therapy. N Engl J Med 365:1673–1683. https://doi.org/10.1056/NEJMoa1106152
Liu Y, Yang Y, Suo Y, Li C, Chen M, Zheng S, Li H, Tang C, Fan N, Lan T, Zhou J, Li Y, Wang J, Chen H, Zou Q, Lai L (2022) Inducible caspase-9 suicide gene under control of endogenous oct4 to safeguard mouse and human pluripotent stem cell therapy. Mol Ther Methods Clin Dev 24:332–341. https://doi.org/10.1016/j.omtm.2022.01.014
Yagyu S, Hoyos V, Del Bufalo F, Brenner MK (2015) An suicide gene to improve the safety of therapy using human induced pluripotent stem cells. Mol Ther 23:1475–1485. https://doi.org/10.1038/mt.2015.100
Shi ZD, Tchao J, Wu L, Carman AJ (2020) Precision installation of a highly efficient suicide gene safety switch in human induced pluripotent stem cells. Stem Cells Transl Med 9:1378–1388. https://doi.org/10.1002/sctm.20-0007
Download references
This study was supported by the National Natural Science Foundation of China (No. 82270846).
Author information
Authors and affiliations.
Molecular Medicine Research Center and State Key Laboratory of Biotherapy, West China Hospital, Sichuan University, 1 Keyuan 4th Road, Gaopeng Street, Chengdu, 610041, China
Xingrong Feng, Hongmei Zhang, Shanshan Yang, Daxin Cui, Yanting Wu, Xiaocun Qi & Zhiguang Su
You can also search for this author in PubMed Google Scholar
Contributions
XRF, HMZ, SSY, DXC, YTW, XCQ and ZGS conceptualization. XRF and ZGS: writing original draft and editing. All authors have read and approved the final manuscript.
Corresponding author
Correspondence to Zhiguang Su .
Ethics declarations
Conflict of interest.
The authors declare no conflict interest.
Ethical approval
This article does not contain any studies with human participants or animals performed by any of the authors.
Additional information
Publisher's note.
Springer Nature remains neutral with regard to jurisdictional claims in published maps and institutional affiliations.
Rights and permissions
Springer Nature or its licensor (e.g. a society or other partner) holds exclusive rights to this article under a publishing agreement with the author(s) or other rightsholder(s); author self-archiving of the accepted manuscript version of this article is solely governed by the terms of such publishing agreement and applicable law.
Reprints and permissions
About this article
Feng, X., Zhang, H., Yang, S. et al. From stem cells to pancreatic β-cells: strategies, applications, and potential treatments for diabetes. Mol Cell Biochem (2024). https://doi.org/10.1007/s11010-024-04999-x
Download citation
Received : 16 January 2024
Accepted : 21 March 2024
Published : 20 April 2024
DOI : https://doi.org/10.1007/s11010-024-04999-x
Share this article
Anyone you share the following link with will be able to read this content:
Sorry, a shareable link is not currently available for this article.
Provided by the Springer Nature SharedIt content-sharing initiative
- Pancreatic β-cells
- β-cell heterogeneity
- Cell reprogramming
- Disease modeling
- Drug discovery
- Cell therapy
- Find a journal
- Publish with us
- Track your research

Institute for Stem Cell & Regenerative Medicine
Disease impact.
Type 1 Diabetes is an autoimmune diseases that occurs when the pancreas stops producing insulin for reasons that are not well understood. People living with Type 1 Diabetes require daily injections of insulin to survive and are at risk of both life-threatening hypoglycemia (low blood sugar) and lifelong health complications. Approximately 1.25 million children and adults in the United Sates have Type 1 Diabetes. An additional 30 million Americans have Type 2 Diabetes, a condition in which the pancreas produces insulin unreliably. The combined national healthcare costs of Type 1 and Type 2 diabetes exceed $320 billion a year.
Current Research
Investigators at the Institute for Stem Cell and Regenerative Medicine (ISCRM) are studying the mechanisms that regulate the development and function of beta cells in the pancreas that produce insulin – a key to future treatments for any type of diabetes. Current research is progressing on several fronts. In several collaborating labs, ISCRM teams are:
- Studying the conditions that make beta cells bind together in pancreatic islets (and therefore secrete more insulin)
- Attempting to coax stem cells to become insulin-producing beta cells
- Testing whether turning off a protein that controls cell proliferation in the pancreas could help beta cells grow
- Modeling islet tissue in dishes to understand the onset of diabetes and help create a pathway to transplantation
Improving Human Health
Behind the multipronged approach to diabetes research is a determination to improve quality of life for millions of people with Type 1 and Type 2 diabetes. Right now at ISCRM, Vincenzo Cirulli MD, PhD, is screening for biological factors that could promote the growth of beta cells necessary for insulin production. Dr. Cirulli’s ISCRM colleague Laura Crisa MD, PhD is using a “disease-in-a-dish” model to study how islet cells falter and whether they can be regenerated, and eventually transplanted, into patients.
Faculty Researchers
Vincenzo Cirulli MD, PhD Laura Crisa MD, PhD
REVIEW article
Advancements in culture technology of adipose-derived stromal/stem cells: implications for diabetes and its complications.
- 1 Department of Endocrinology, Union Hospital, Tongji Medical College, Huazhong University of Science and Technology, Wuhan, China
- 2 Hubei Provincial Clinical Research Center for Diabetes and Metabolic Disorders, Wuhan, China
Stem cell-based therapies exhibit considerable promise in the treatment of diabetes and its complications. Extensive research has been dedicated to elucidate the characteristics and potential applications of adipose-derived stromal/stem cells (ASCs). Three-dimensional (3D) culture, characterized by rapid advancements, holds promise for efficacious treatment of diabetes and its complications. Notably, 3D cultured ASCs manifest enhanced cellular properties and functions compared to traditional monolayer-culture. In this review, the factors influencing the biological functions of ASCs during culture are summarized. Additionally, the effects of 3D cultured techniques on cellular properties compared to two-dimensional culture is described. Furthermore, the therapeutic potential of 3D cultured ASCs in diabetes and its complications are discussed to provide insights for future research.
1 Introduction
Stem cell-based therapy, including pluripotent stem cells (PSCs) and mesenchymal stromal/stem cells (MSCs), represents an innovative therapeutic strategy that capitalizes on the distinctive characteristics of stem cells, such as self-renewal and differentiation capabilities, to facilitate the regeneration of impaired cells and tissues within the body or the substitution of these cells with new, healthy, and fully functional cells by delivering exogenous cells ( 1 ).
PSCs are characterized as a type of self-renewing cells capable of differentiating into diverse cellular phenotypes originating from the three germ layers of the body ( 2 ). PSCs, including embryonic stem cells (ESCs) and induced pluripotent stem cells (iPSCs), has revolutionized stem cell research and cell-based therapy ( 3 ). Nonetheless, the utilization of ESCs is constrained by ethical considerations, the possibility of immunological rejection, and the potential for tumorigenicity ( 1 , 4 ). In contrast, iPSC technology overcomes ethical dilemmas associated with ESCs derived from human embryos, enabling the creation of patient-specific pluripotent stem cells. However, iPSCs are generated through the ectopic expression of pluripotency factors, often facilitated by viral vectors or non-viral reprogramming factors, which may lead to genomic instability ( 5 , 6 ). Besides, iPSCs have been shown to elicit T cell-dependent immune response ( 7 ) and promote tumor formation ( 3 , 8 ). Consequently, thorough safety assessments are imperative prior to iPSC transplantation.
Mesenchymal stromal/stem cells (MSCs) are adult stem cells with multipotent capabilities, including self-renewal (albeit limited in vitro) and differentiation into various mesenchymal lineages ( 9 , 10 ). MSCs have been shown to overcome ethical concerns and mitigate the risk of mutational side effects associated with. Additionally, MSCs exhibit the lowest immunogenicity compared to other stem cell types, making them a favorable option for clinical use ( 11 ). In the field of organ and cell transplantation, MSCs have been utilized for their secretion of growth factors and immunoprotective cytokines. Their ability to differentiate into various cell types has been harnessed for applications in tissue engineering ( 12 ). Among these, adipose-derived MSCs (ASCs) are particularly advantageous due to their larger storage with less discomfort and damage to the donor site, easier accessibility without significant donor site morbidity, higher proliferation ability, fewer ethical concerns, and fewer immunological rejection ( 11 , 13 , 14 ). Furthermore, some growth factors and immunomodulators are more actively secreted in ASCs ( 13 ). Therefore, ASCs may be a better candidate for clinical application in theory.
Diabetes mellitus (DM) is a severe and chronic disease characterized by elevated blood glucose levels resulting from aberrant islet β-cell biology and insulin action ( 15 ). In 2021, the global population living with diabetes reached 529 million ( 15 ). Given β-cell dysfunction across various types of DM, most patients ultimately require insulin therapy ( 16 – 18 ). However, this therapy is frequently limited by individual factors, such as weight gain, fear of needles and lifestyle considerations, all of which contribute to poor glycemic control. Furthermore, insulin therapy cannot reverse β-cell damage and progress of diabetes, or replicate the normal physiological state. In recent clinical applications, pancreatic islet and cell transplantation have emerged as potential strategies ( 19 ). However, these procedures have numerous challenges, including the scarcity of suitable donors, surgical complexities, side effects associated with immunosuppressive agents as well as exhaustion of transplanted organs and cells ( 11 ). Furthermore, it is necessary to maintain β-cell function and blood glucose homeostasis, otherwise life-threatening complications are likely to occur ( 20 ).
In the treatment of diabetes and its complications, ASCs have been used due to their inherent attributes such as self-renewal capacity, differentiation potential, homing mechanism and immunosuppressive property ( 11 , 21 ). Furthermore, three-dimensional (3D) cultured cells are studied to prolong the lifespan of transplanted cells and enhance their pro-healing functions in unfavorable environments ( 22 – 25 ). Recent literature provides numerous strategies for obtaining 3D cultured ASCs ( 26 ). These cells possess enhanced abilities to maintain their stemness and display multilineage plasticity compared to cells cultured in adhesion ( 26 ). Moreover, 3D cells more closely mirror biological processes compared to cells cultured in traditional monolayers, driving the need for the development of 3D culture, including spheroids, organoids, organ-on-a-chip models, and bioprinting ( 27 – 29 ).
Despite being an emerging and rapidly developing technology, there is currently no standardized method for ASC culture and no summary for the research of 3D cultured ASCs in diabetes and its complications. In this review, we summarize current knowledge about monolayer ASC culture techniques, with a particular emphasis on the influential factors during culture. Additionally, the effects for cellular properties of 3D cultured methods compared to two-dimensional (2D) culture is described. Furthermore, the therapeutic potential of 3D cultured ASCs in diabetes and its complications are discussed to provide insights for future research.
2 Nomenclature of adipose-derived stromal/stem cells
There is inconsistency in the nomenclature of this plastic adherent cell population isolated from adipose tissue ( 30 ). In 2006, the International Society for Cellular Therapy (ISCT) acknowledged the “inconsistencies and ambiguities” of the term “mesenchymal stem cells” and recommended a new designation: multipotent mesenchymal stromal cells ( 31 ). It is recommended to use the abbreviation “MSCs” in conjunction with extra information like AD-MSCs ( 9 ) (adipose tissue-derived MSCs) or MSC(AT) ( 32 ) and clearly define stem cells or stromal cells in terms of their function ( 9 ). Additionally, Caplan proposed the term “medicinal signaling cells” due to their therapeutic actions, which include homing to the site of injury and secreting regenerative and immunomodulatory factors ( 33 ). Despite the advocacy for standardization in nomenclature, it is still most common to refer to MSCs as “mesenchymal stem cells”, followed by “mesenchymal stromal cells” or a combined use of “stem/stromal” terms ( 34 ). In this review, following search terms for this kind of cells were adopted: “adipose-derived stromal cells”, “adipose-derived stem cells”, “adipose-derived stromal/stem cells”, “ASCs” and “ADSCs”, and having no limitation to the human or animal species.
3 Monolayer culture techniques
In the 1960s, Rodbell and Jones pioneered the initial method of isolating cells from adipose tissue ( 35 – 37 ). The researchers isolated stromal vascular fraction (SVF) from rat fat pads, which contained heterogeneous cells. In the final step, adherent plastic cells within the SVF were selected and enriched for “preadipocytes”. In 2001, ZUK et al. obtained a fibroblast-like cell population or a processed lipoaspirate from human lipoaspirate. They determined these cells could differentiate into adipogenic, osteogenic, chondrogenic, and myogenic cells in vitro, which opened up new avenues for MSC research ( 38 ). The isolation and culture process of ASCs is shown in Figure 1 .
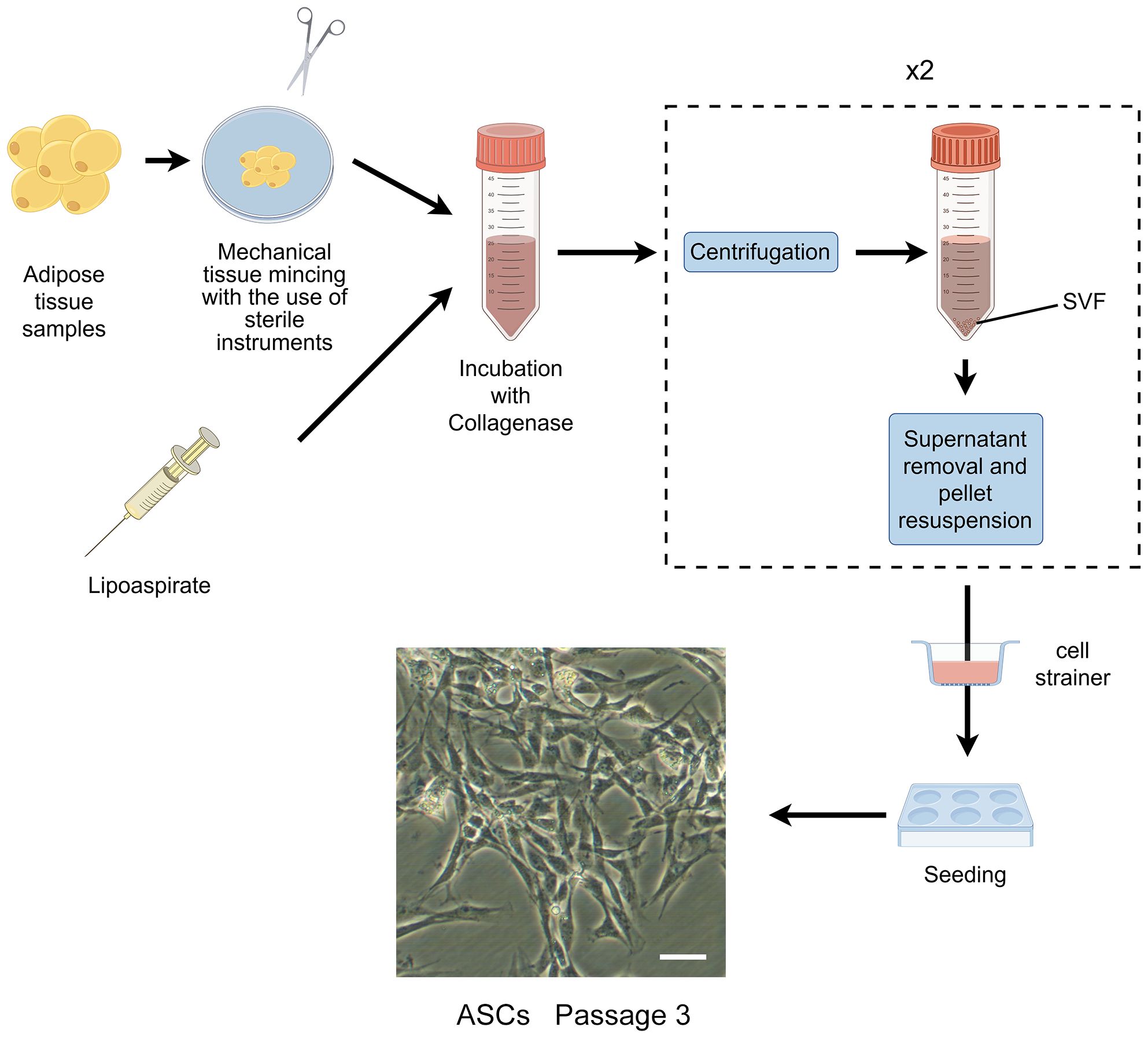
Figure 1 The isolation process of ASCs. The cells showed are isolated from rat’s inguinal adipose tissue. Scale bar, 200μm. SVF, stromal vascular fraction; ASCs, adipose-derived stromal/stem cells.
The characterization of ASCs involves fulfilling specific criteria related to cellular morphology ( 39 , 40 ), immune-phenotypic ( 10 ), and differentiation capacity ( 10 , 31 ). As high quality of cells is the prerequisite for their application, various factors that may influence their biological functions during culture have been proposed ( Figure 2 ).
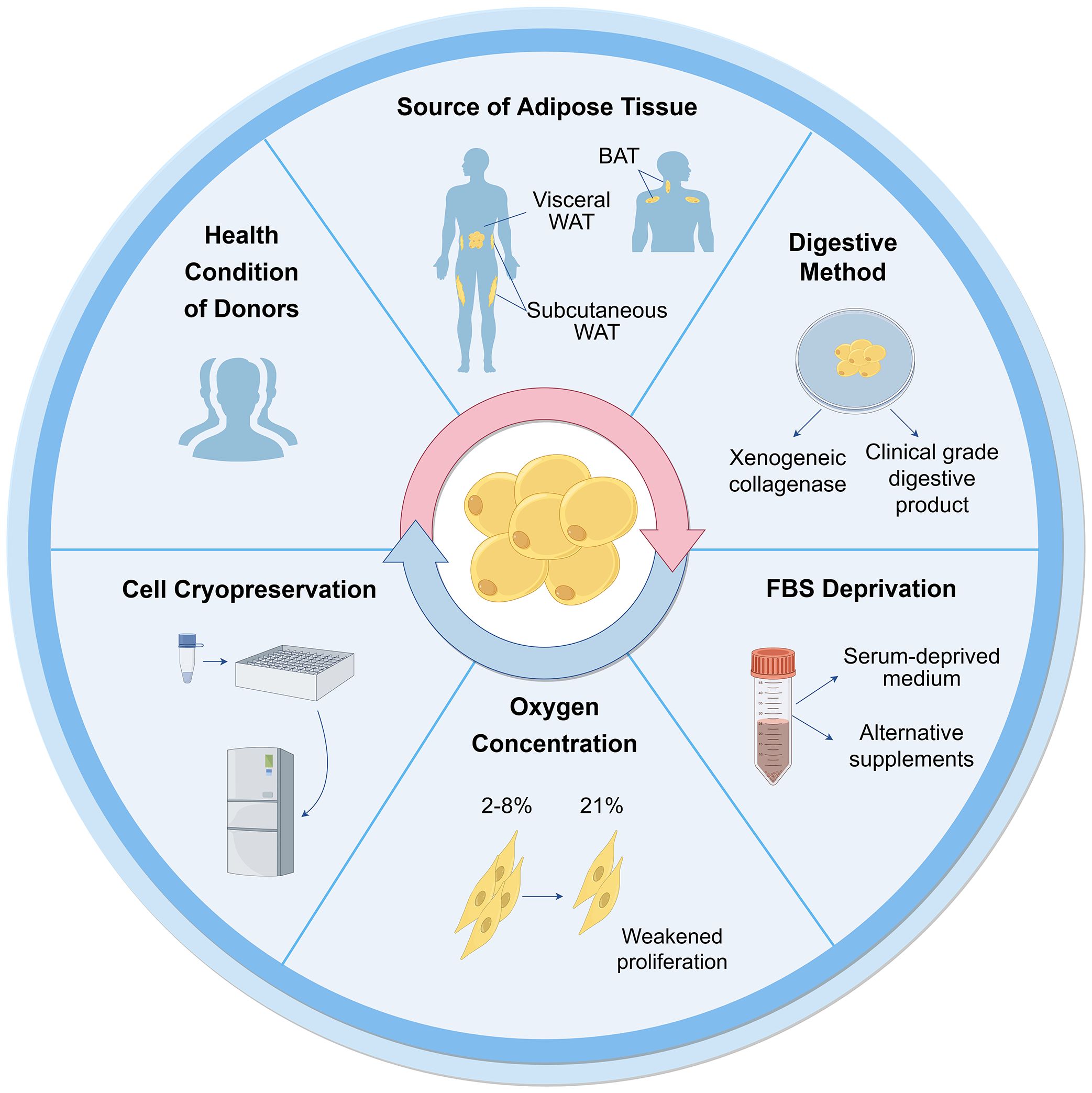
Figure 2 Influential factors on biological functions of ASCs during culture. Many aspects are reported to influence ASC culture and their biological functions. These can broadly be divided into the sources of tissues and cells, techniques of isolation, culture and cryopreservation. WAT, white adipose tissue; BAT, brown adipose tissue; FBS, fetal bovine serum.
3.1 Tissue and cell sources
3.1.1 health conditions of donors.
Cells can be obtained from healthy donors or individuals with varying degrees of diabetes, obesity, and other chronic diseases. The use of autologous and allogeneic ASCs should be carefully considered. Autologous cells have advantages in terms of histocompatibility and infectious concerns ( 41 ), but their functionality may be compromised in an unhealthy environment. ASCs derived from diabetic donors have shown reduced proliferation ability and paracrine activity compared to autologous ASCs from healthy individuals ( 42 – 44 ), but they still hold potential in cell therapies ( 45 – 47 ). Additionally, Obesity has an adverse impact on ASCs, resulting in defective functionalities and properties ( 48 ). ASCs from individuals with obesity exhibit decreased telomerase activity and telomere length ( 49 ). There are no significant differences observed in ASCs between oncological patients and healthy subjects ( 50 , 51 ). However, ASCs from donors exposed to radiotherapy and chemotherapy exhibit altered cell migration, proliferation, and differentiation capacity ( 48 ). The outcomes are also correlated with other demographics, such as age, gender, and ethnicity ( 52 ).
3.1.2 Types of adipose tissue
White adipose tissue (WAT) mainly exists in two types: subcutaneous and visceral adipose tissue. ASCs obtained from subcutaneous (S-ASCs) and visceral adipose tissue (V-ASCs) share similar cell viability and surface markers but differ in motility, secretory function, and expression of stemness-related genes ( 53 ). However, S-ASCs have a greater differentiation capacity to adipogenic and osteogenic cells, and V-ASCs proliferate slower, require stronger stimulation for differentiation ( 54 ), and secrete higher levels of inflammatory cytokine such as interleukin (IL)-6, IL-8 and tumor necrosis factor (TNF)-α ( 55 ). Wada et al. ( 56 ). also found that V-ASCs and S-ASCs release inflammatory and angiogenesis cytokines differently. Moreover, ASCs in the superficial layer, located closer to the dermis, exhibited hyperplastic and angiogenic capacities, while ASCs in the deep layer were characterized by inflammatory properties similar to V-ASCs ( 27 ).
Furthermore, studies have shown the presence of ASCs derived from brown adipose tissue (BAT) ( 57 , 58 ). The characteristics of ASCs derived from BAT differ from those of WAT, particularly, the expression of myogenic factor 5 (Myf5) and myogenic origin. In these cells, gene expression profiles are unique, particularly the higher expression of genes associated with BAT including uncoupling protein-1 (UCP1), peroxisome proliferator-activated receptor gamma coactivator 1-alpha (PGC-1α), PR domain containing 16 (PRDM16), and CAMP responsive element binding protein one (CREB1). Therefore, the tissue and cell sources should be considered for further application.
3.2 Isolation and culture
3.2.1 collagenase digestion.
The first crucial step in obtaining cells from adipose tissue is cell isolation. Currently collagenase digestion remains the most common method to obtain cells due to its simplicity and high cell purity ( 41 , 48 ). However, the use of xenogeneic collagenase may lead to pathogen transmission and immune response in vivo. To be considered safe, the development of clinical grade digestive products is crucial for the isolation of ASCs. Carvalho et al. demonstrated that several alternative enzyme products, including Collagenase NB 4 Standard Grade (NB4) [Serva], Collagenase Type 1 (CLS1) [Worthington], Collagenase (Animal Origin Free)-A (CLSAFA) [Worthington], and Liberase [Roche], were equally effective as research-grade products ( 59 ). Kølle et al. implemented a clinical trial using cells which were isolated by clinical collagenase NB 6 ( 60 ).
3.2.2 Serum deprivation
Fetal bovine serum (FBS) is another important consideration for ASC culture and application, similar to xenogeneic collagenases. The available studies showed that human ASCs (hASCs) maintain their stemness in serum-deprived medium ( 61 ). In the absence of FBS for 48 hours, hASCs showed reduced metabolism and proliferation, but maintained the expression of crucial surface markers, without undergoing apoptosis or necrosis ( 51 ). Human ASCs cultured in STK2 (a chemically-defined serum-free medium) exhibited enhanced proliferation, elevated expression of MSC surface markers, and diminished cell aging compared to those cultivated in media supplemented with FBS ( 62 ). According to these observations, FBS deprivation does not cause impacts that would prevent cellular clinical application.
Other alternative supplements have been investigated as potential substitutes for FBS. Human platelet lysates (HPLs) could serve as a superior supplement. They were found to augment the proliferative capacity of hASCs in comparison to FBS, while simultaneously preserving their untransformed state and differentiation ability ( 63 , 64 ). Kocaoemer et al. observed that hASCs cultured in medium supplemented with either thrombin-activated platelet rich plasma (tPRP) or pooled human serum (HS) exhibited similar properties, although a reduction in adhesion was observed in cells cultured in tPRP-supplemented medium ( 65 ). According to the whole genome gene analysis, 90 genes were significantly expressed more in hASCs cultured in FBS-supplemented medium ( 66 ).
3.2.3 Oxygen concentration
As the oxygen concentration of adipose tissue in vivo is 2%-8%, ASCs exist in a relatively low-oxygen microenvironment ( 67 , 68 ). However, most ASCs are cultured under normal oxygen conditions (21% oxygen concentration) in vitro. Human subcutaneous ASCs cultured in hypoxic conditions in vitro exhibited increased proliferation rates and secretion of growth factors ( 69 ). Tirza et al. discovered weakened proliferation ability, increased accumulation of reactive oxygen species (ROS), and genetic instability of rat visceral ASCs cultured under normal oxygen experienced, which could be improved by lowering the culture temperature ( 67 ).
3.2.4 Cell cryopreservation
Despite the diminished cell viability and lower colony-forming-unit percentages observed in cells derived from cryopreserved lipoaspirate compared to fresh lipoaspirate-derived cells, the viable cells that remained exhibited preserved adhesive and proliferative properties ( 70 ), which could counteract the negative effect with continued cell growth ( 71 ). After prolonged cryopreservation at 70°C, the number of viable cells decreased as well as their viability ( 71 ). A cryopreservation medium containing HS, HS albumin, or knockout serum replacement did not affect the gene expression, differentiation ability, and immunophenotype of hASCs for a duration of 3-4 freeze-thaw cycles, but significantly reduced the proliferation. Thus, it has been recommended that cells for clinical application should not undergo more than two freeze-thaw cycles ( 72 ).
In summary, isolation and culture methods can affect ASCs properties, therefore, there is still a need to look for appropriate culture protocol that will provide the right number and characteristics of ASCs without affecting their therapeutic potential for clinical application.
3.3 Cellular senescence and potential interventions
Cellular senescence, also called aging, has always been an obstacle to the development of MSC therapy. Some studies confirmed the stability of ASCs during a certain period (usually up to the sixth or seventh passage) ( 51 , 73 , 74 ). However, Yin et al. found that hASCs rapidly underwent replicative senescence and lost stem cell properties over 21 days by current 2D culture ( 75 ). During long-term culture, senescent cells experience a cessation in proliferation, and exhibit distinct morphological and physiological features, including enlarged nuclear and cytoplasmic volumes, heightened β-galactosidase enzyme activity, decreased expression of β cell-specific Moloney murine leukemia virus integration site 1 (Bmi-1), telomere shortening and accumulation of ROS ( 76 , 77 ). The stability and safety of ASCs should be considered in application, thus, many research efforts have been enhanced to address the problem of cellular aging.
Immortalization techniques have been shown to overcome senescence in primary cells ( 78 ). Kang et al. discovered that ectopic expression of telomerase reverse transcriptase (TERT) in non-human primates ASCs enabled cells to maintain proliferative potential and multipotent differentiation ability ( 79 ). Tchkonia et al. generated preadipocyte strains from single abdominal subcutaneous, mesenteric and omental human preadipocytes through stable expression of human TERT (hTERT). These strains were capable of repeated subculturing and maintained the capacity for differentiation, as well as the specific dynamic characteristics of fat depot cells ( 80 ). Wolbank et al. found hTERT overexpression generated ASC lines (ASCs hTERT ) exhibited continuous growth and showed minimal changes in morphology, surface marker profile, karyotype, immunosuppressive capacity and differentiation potential ( 81 ). Similarly, Shamsi and Tseng developed protocols for immortalizing brown and white preadipocytes ( 82 ). Furthermore, researchers have cultured ASCs with TERT expression to conduct further researches in regenerative medicine and other medical fields ( 79 , 83 – 85 ).
Furthermore, Tátrai et al. found that human ASCs hTERT and ASCs generated by the co-transduction of hTERT and Bmi-1 retained MSC features and did not senesce, whereas ASCs generated by the overexpression of Bmi-1 exhibited limited replicative potential. Notably, a subpopulation of ASCs hTERT also acquired aberrant karyotype and showed signs of transformation after long-term culture ( 86 ).
However, Balducci et al. found that hTERT alone failed to immortalize hASCs. Moreover, hASCs that were co-transduced with hTERT and human papillomavirus (HPV)-E6/E7 were successfully immortalized and could secrete significant amount of hepatocyte growth factor (HGF) and vascular endothelial growth factor (VEGF), albeit with reduced differentiation properties and some chromosomal aberrations ( 87 ). Darimont et al. demonstrated that co-transduction of hTERT and HPV-E7 enabled human preadipocytes to extend their lifespan and maintain their capacity for differentiation ( 88 ).
The overexpression of simian virus 40 large T antigen (SV40T) has been widely employed as a strategy to overcome replicative senescence in human primary cells. However, it was found that the adipogenic differentiation process was blocked by SV40T expression in 3T3-F442A cells ( 89 ). Human ASC lines operated by co-transduction of hTERT and SV40T underwent chromosome aberration, deviated from the normal MSC phenotype, and lose the ability of differentiate ( 86 , 87 ).
Although there were variations in results across different studies, it is generally established that cell immortalization can be achieved through gene editing technology ( Table 1 ). Notably, the possibility of karyotype variation should be taken into consideration in these immortalized cells constructed by gene editing technology.
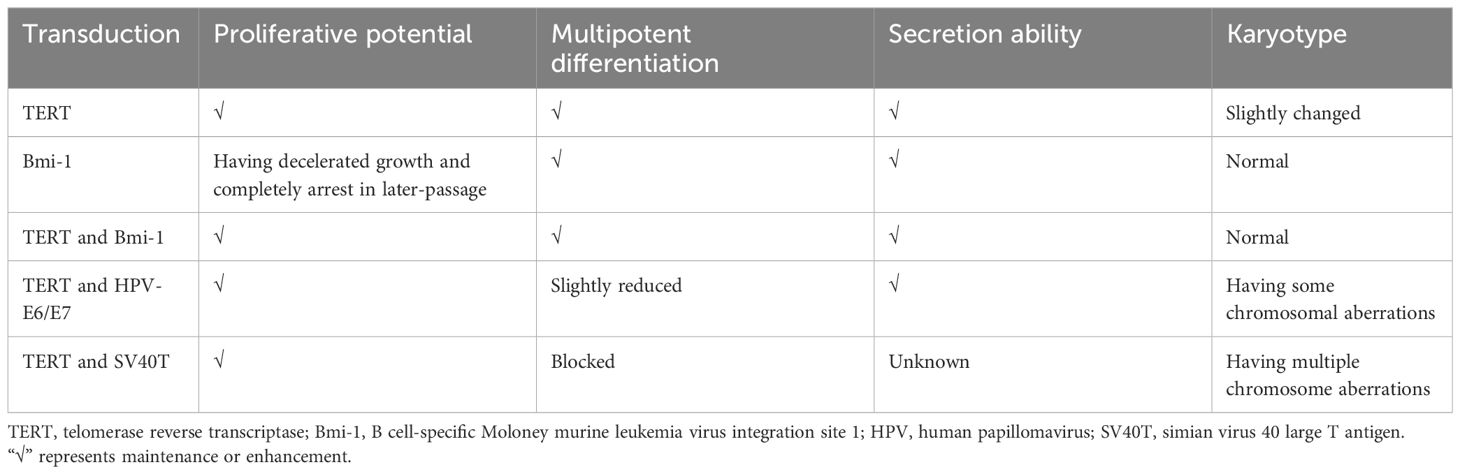
Table 1 Immortalization of ASCs through gene editing technology.
4 3D culture techniques
Significantly, advancements in stem cell and 3D culture technologies have enabled the creation of cellular models that accurately mimic the histological, molecular, and physiological characteristics of tissues and organs ( 29 ). The formation of 3D cultures relies on the self-organization and differentiation of cells, as well as signaling cues from the extracellular matrix (ECM) and conditioned media ( 90 ).
4.1 Cell types
3D cultures are typically self-assembled in vitro 3D structures derived from primary tissues or various types of stem cells, including MSCs, iPSCs, and ESCs. Various cell types exhibit distinct developmental pathways, underscoring the importance of selecting an appropriate initial cell population for the successful establishment of organoid cultures. 3D culture of ESCs has not been a priority due to their ethical concerns. 3D culture models derived from MSCs have been shown to highly recapitulate the homeostasis and regenerative capacity of the tissue of origin ( 91 ). Conversely, models derived from iPSCs often hardly recapitulate the adult tissue stage, instead resembling the fetal tissue stage ( 92 , 93 ). 3D culture models derived from ASCs are generated without genetic modification by transcription factors, unlike those derived from iPSCs ( 94 ). Moreover, ASC exhibit immune privileged properties, and accordingly show excellent safety for allogeneic transplantation in multiple human clinical trials ( 4 ). Therefore, ASCs is a cell type with great potential and advantages in 3D culture technology.
4.2 Effects of culture techniques on cellular properties
Despite numerous studies, there is no standardized method for 3D ASC culture. It is necessary to comprehend the impact of different 3D culture techniques on cellular properties in contrast to traditional 2D culture.
4.2.1 Cell viability and stemness
The stemness properties of MSCs are retained in the in vivo microenvironment, which includes soluble growth factors, cell-cell interactions and cell-matrix interactions ( 95 , 96 ). Increasing evidence has indicated that the cellular microenvironment significantly influences stemness properties ( 95 , 97 ). In comparison to conventional monolayer cultures, 3D cultured methods provide a cellular niche that more closely resembles the in vivo microenvironment ( 98 ).
Existing techniques for ASC culture can be categorized into scaffold-free and scaffold systems ( 26 , 99 ). The conventional scaffold-free culture techniques, such as the use of low adhesion plates, hanging drops, and spinner flasks, have been shown to impact the viability and stemness of ASCs.
Low adhesion plate culture method involves the formation of spheroids by suspending cells on a surface with low adhesion properties. Guo et al. successfully generated 3D spheroids using non-adhesive agarose Petri dishes. This method was found to overcome poor post thaw cells and improve the viability and neural differentiation potential of hASCs ( 100 ). Similarly, Coyle et al. conducted a study examining hASC spheroids with various sizes and demonstrated the enhanced viability of spheroids was achieved through anaerobic glycolysis in conditions of increased glucose availability and decreased oxygen levels ( 101 ). Di Stefano et al. conducted a comparative analysis of hASCs cultured in ultralow culture flasks and hASCs with 2D primary cultures. Their study identified distinct molecular expression patterns of genes associated with stemness, as well as genes related to anti-aging, oxidative stress, and telomeres maintenance of hASCs ( 102 ). Rybkowska et al. conducted a study in which 3D hASC spheroids were cultured using antiadhesive plates. However, they observed that the spheroids exhibited slightly lower viability, reduced proliferation rates, but higher expression of stemness-related transcriptional factors compared to cells cultured in monolayer. Additionally, the 3D culture resulted in increased mitochondrial DNA content, oxygen consumption rate, and extracellular acidification rate. Elevated levels of ROS and decreased intracellular lactic acid levels were also detected ( 103 ).
The hanging drop method technique capitalizes on the intrinsic tendency of cells to self-assemble into three-dimensional aggregates needless of scaffolding. A drop is formed within an inverted plate and held in place due to surface tension. Jin et al. utilized the hanging-drop technique to produce hASC microtissues in a smooth muscle inductive medium supplemented with human transforming growth factor β1, and subsequently bioprinted these induced microtissues onto a 3D framework. The microtissues retained their phenotypic characteristics post-bioprinting. Cell viability and proliferation within the 3D microtissues were consistently superior in comparison to the traditional single-cell bioprinting approach ( 104 ).
The spinner flask facilitates the generation of fluid flow, which discourages cellular adhesion and facilitates cellular aggregation. Bangh et al. placed hASC spheroids in spinner flasks under 1% oxygen. The spheroids exhibited faster growth rates compared to monolayer cultures. Additionally, they observed an upregulation of survival factors in response to the spheroid size ( 105 ).
Another commonly employed approach involves seeding stem cells into scaffolds that mimic the ECM of native tissues, which can be fabricated using biologically derived or synthetic materials. Natural scaffolds consist predominantly of collagen, fibrin, gelatin, vitronectin, laminin, alginate, hyaluronic acid (HA), or decellularized materials, while synthetic scaffolds may consist of materials such as polyesters, polyethers, polyethylene glycol, and polylactic acid (PLLA) ( 106 ). Several studies have investigated the use of different hydrogels to create ASC spheroids, utilizing commonly used materials in tissue engineering such as HA and chitosan, resulting in enhanced stemness gene expression compared to traditional adhesion plate cultures ( 107 – 110 ). A poly(ethylene glycol) (PEG) hydrogel microwell pattern was fabricated on a poly(N-isopropylacrylamide) hydrogel substrate to regulate the size of spheroids. The viability of hASC spheroids exceeded 97.5% ( 111 ).
Based on mechanical structure or new systems, various novel techniques were devised to create 3D ASC structures with enhanced viability, increased stemness, and enhanced differentiation capabilities, such as the following: A switchable water-adhesive, super-hydrophobic nanowire surface ( 112 ); microgravity bioreactors ( 113 ); microwell plates employed with gelatin microparticles ( 114 ); microfabricated porous tissue strands (pTSs) ( 115 ); a method defined “all-in-one platform” with hydrogels with an embossed surface (HES) ( 116 ); gelatin hydrogels with microbial transglutaminase (mTG) ( 117 ); and TeSR-E8 medium (a highly chemically defined medium) in conventional tissue culture polystyrene dishes ( 118 ). Furthermore, Labriola et al. utilized polymer-based, cell mimicking microparticles (CMMPs) to deliver distinct, stable mechanical cues to hASCs in 3D spheroid culture. Mechanically tuned CMMPs controlled whole-spheroid mechanical phenotype and stability but minimally affected differentiation response ( 119 ).
Based on the findings of these studies, it is evident that the majority of research indicates that 3D culture enhances cell viability, stemness, proliferation rate, and metabolic functions, with only a few exceptions showing a decrease in cell viability.
4.2.2 Differentiation ability
Multilineage differentiation potential of ASCs towards both mesenchymal and non-mesenchymal lineage cells have been reported, particularly towards adipogenic, chondrogenic, and osteogenic lineages, which can be facilitated by the introduction of lineage-specific factors ( 120 ).
Adipogenic differentiation: Decellularized adipose tissue (DAT) based hydrogels have been demonstrated to closely replicate the native ECM environment, effectively inducing adipogenic differentiation and promoting the proliferation of hASCs ( 121 , 122 ). In a study by Zhang et al., hASC spheroids cultured in a microgravity bioreactor exhibited enhanced stemness properties and adipogenic differentiation potential compared to monolayer culture ( 113 ). Hoefner et al. cultured hASC spheroids in growth cell media under agitation at 50 revolutions per minute. After a brief 2-day induction period for adipogenic lineages, it was observed that ASC spheroids exhibited enhanced differentiation capacity within their own ECM when compared to traditional 2D cultures ( 123 ). These findings suggest that utilizing 3D ASC culture may be a promising approach for adipose tissue engineering applications.
However, Rumiński et al. reported that hASC spheroids seeded in 96-well sterile round-bottom culture plates and subjected to gentle rotation on a rotary shaker displayed reduced adipocyte differentiation ( 124 ). The elastin-like polypeptide (ELP)-polyethyleneimine (PEI) coated surface was demonstrated a suitable cell culture material ( 125 ). However, the study conducted by Turner et al. revealed that triglyceride accumulation was less pronounced in hASC spheroids seeded on ELP-PEI coated surfaces compared to 3T3-L1 adipocytes, correlated with smaller average spheroids, suggesting a relatively slower differentiation process ( 126 ).
Chondrogenic differentiation: Yoon et al. employed the spinner flask method to illustrate that 3D hASC spheroids exhibit enhanced chondrogenic capabilities when cultured in a specific differentiation medium as opposed to monolayer culture ( 127 ). Tsai et al. employed mTG, an enzyme with high specificity across a broad temperature range, to crosslink gelatin. The evaluation of differentiation potential revealed that hASC spheroids within the 3D gelatin/mTG hydrogel demonstrated heightened activity, particularly in adipogenesis and chondrogenesis, in comparison to the cell suspension group ( 117 ). Furthermore, when comparing hASC spheroids cultured using microwell techniques to ASCs cultured in a 2D monolayer, it was observed that cell survival and chondrogenic potential were enhanced, while apoptosis was diminished. Injecting hASC spheroids exerted enhanced regenerative capabilities for articular cartilage and effectively halted the advancement of surgically induced osteoarthritis through the paracrine mechanism of action, when compared to ASCs in single-cell suspension ( 128 ).
Osteogenic differentiation: Gurumurthy et al. illustrated that 3D hASCs cultivated on ELP-PEI scaffolds exhibited a heightened propensity for differentiation towards the osteogenic lineage in comparison to 2D cultures ( 129 ). Human ASCs were cultured in 3D systems devoid of bioactive material components: spheroids and polystyrene scaffolds. Alkaline phosphatase activity, a marker of early osteogenesis, exhibited increased levels in ASC spheroids and ASC-seeded scaffolds in comparison to 2D cultures. The expression of the osteoblast marker, including Runt-related transcription factor 2, and osterix and integrin binding sialoprotein was significantly up-regulated in spheroids compared to polystyrene scaffolds and 2D culture ( 124 ). Kim et al. conducted a study to evaluate the osteogenic potential of hASCs in 2D and 3D culture environments. Through comprehensive analysis of transcriptome sequencing data, they identified an upregulation of genes associated with skeletal development, bone formation, and bone remodeling processes in hASCs cultured in concave microwells ( 130 ).
Differentiation into other lineages: Cheng et al. utilized chitosan films to form hASC spheroids, which, when cultured in appropriate induction media, exhibited enhanced differentiation capabilities, including differentiation into neuron and hepatocyte-like cells ( 131 ). Guo et al. observed an increased capacity for neural differentiation in 3D hASC spheroids cultured in agarose 3D Petri dishes ( 100 ). Amirpour et al. employed a defined neural induction medium with small molecules to directly differentiate hASCs into anterior neuroectodermal cells using hanging drop protocols ( 132 ). Additionally, Salehi et al. conducted a comparison between two differentiation protocols for the generation of retinal precursor-like cells in vitro: hASCs monolayer culture and hanging drop culture with a defined medium. The study indicated that the hanging drop method led to an enhanced yield of retinal precursor differentiation, resulting in precursor-like cells that exhibited responsiveness to the glutamate neurotransmitter ( 133 ). Moreover, the hanging drop method was found to enhance the efficiency of hASC smooth muscle differentiation and improve cell viability within a 3D bioprinted structure ( 104 ). Bagheri-Hosseinabadi et al. observed a higher rate of cardiomyogenic differentiation in hASCs cultured in a 3D hanging drop system with 5-azacytidine compared to the 2D culture ( 134 ).
These findings suggest that the 3D environment may offer enhanced stimuli for the differentiation of ASCs into various lineages. These results have implications for the development of protocols for preparing ASCs for use in clinical studies focused on regeneration.
4.2.3 Paracrine secretion
The paracrine secretion of cytokines such as angiogenic factors, adipokines, neurotrophic factors, and interleukin plays a crucial role in the therapeutic application of ASCs by promoting tissue regeneration and repair ( 120 ).
3D cultured ASCs possess distinct and inherent characteristics independent of the method of formation. The size of 3D cultured ASCs is a critical factor, as larger cells exhibit higher levels of hypoxic factors that stimulate angiogenesis and antiapoptotic gene expression ( 26 ). small spheroids of average spherical shape were generated in 96-well plates. The 3D condition of the hASCs was found to be correlated with elevated levels of VEGF-A and IL-8 expressions in relation to wound healing ( 135 ). Kim et al. introduced HES as a comprehensive platform capable of facilitating the rapid formation and cultivation of a substantial quantity of size-adjustable 3D hASC spheroids. Notably, HES-derived spheroids exhibited a higher VEGF secretion compared to spheroids cultured on a commercially available low-attachment culture plate. Utilizing these advantages, HES-based spheroids were employed for 3D bioprinting, resulting in enhanced retention and VEGF secretion within the 3D-printed construct compared to a similar structure containing single cell suspension ( 116 ). Yu et al. utilized agarose microwells to seed hASCs, generating uniform cell spheroids with adjustable size, and stimulated ECM deposition through the use of ascorbic acid 2-phosphate to form ASC sheets. Transcriptome sequencing analysis indicated upregulation of angiogenesis-related genes in ASC spheroids compared to monolayer ASCs. The study illustrated the stimulatory impact of spheroid formation on ASCs towards endothelial lineage by observing increased expression of cluster of differentiation (CD) 31, which persisted following the seeding of ASC spheroids on cell sheets. Furthermore, compared to ASC sheets, ASC spheroid sheets exhibited heightened expression of VEGF and HGF, and the conditioned medium from ASC spheroid sheets significantly promoted tube formation of endothelial cells in vitro ( 136 ).
Seo et al. innovatively created a switchable water-adhesive, super-hydrophobic nanowire surface to enhance cell-cell and cell-matrix interaction, leading to improved cell viability and paracrine secretion of VEGF in hASC spheroids. The size of hASC spheroids can be easily manipulated on this surface. Accordingly, the spheroids generated on this surface demonstrate significantly heightened angiogenic effectiveness in comparison to spheroids produced through traditional methods such as spinner flask suspension culture and hanging drop culture on a petri dish ( 112 ). The successful establishment of a 3D co-culture model utilizing HA gel and a 10:1 ratio of late-passage hASCs and endothelial colony-forming cells resulted in increased secretion of cytokines, including HGF, VEGF, and epidermal growth factor (EGF), compared to single-cell 3D culture or monolayer culture ( 109 ). These findings suggest potential applications of 3D strategies in angiogenesis and regeneration therapies.
Furthermore, Zhang et al. utilized a low-adhesion cell culture plate to generate rat ASCs (rASCs) into microtissues in vitro. They employed grafts composed of microtissues and polycaprolactone nerve conduit for the purpose of repairing sciatic nerve defects in rats. Their study revealed that microtissues promote the secretion of nerve regeneration-related cytokines, including brain-derived neurotrophic factor, and nerve growth factor, the angiogenic factor such as VEGF, as well as anti-inflammatory cytokines such as IL-4, IL-10, and IL-13. This secretion ultimately facilitated the growth of axons when compared to an equivalent number of cells cultured in a 2D manner ( 137 ). Zhou et al. utilized a hanging drop method to generated murine ASCs-based microtissues, which were subsequently injected into streptozotocin (STZ)-induced diabetic rats for the treatment of erectile dysfunction. The findings demonstrated elevated expression of VEGF, nerve growth factor, and TNF-stimulated gene-6 within the microtissues, indicating neuroprotective and anti-inflammatory properties ( 138 ).
Overall, the use of specific culture media and 3D cultured techniques can enhance the differentiation potential and paracrine secretion of ASCs. Hence, it is imperative to carefully deliberate on the selection and refinement of techniques for producing 3D cultured ASCs, as they have the potential to impact the characteristics of cells.
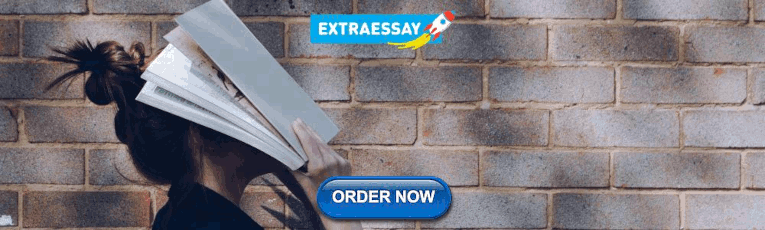
5 The potential of 3D cultured ASCs for diabetic therapy
Diabetes as a multi-organ disease, is a significant cause of increased morbidity and mortality worldwide. In the treatment of diabetes and its complications, ASCs have been used due to their inherent attributes such as self-renewal capacity, differentiation potential, homing mechanism and immunosuppressive property ( 11 , 21 , 139 ). Currently, the clinical trials of ASCs for treating diabetes and its associated complications, including the diabetic foot ulcer (DFU), diabetic critical limb ischemia, and diabetic nephropathies, are still in the preliminary research stage ( Table 2 ). There is a lack of agreement regarding the optimal method of administration to achieve enhanced therapeutic outcomes. Potential routes of administration include intravascular injection, local tissue injection, and thymus injection. In diabetic patients, the most commonly used administration routes are intraportal injection and intravenous infusion ( 140 , 141 , 143 , 144 ). For diabetic angiopathy conditions like DFU, common delivery methods include local injection of ASCs and direct application of 3D ASC grafts onto the wound site ( 145 – 147 ).
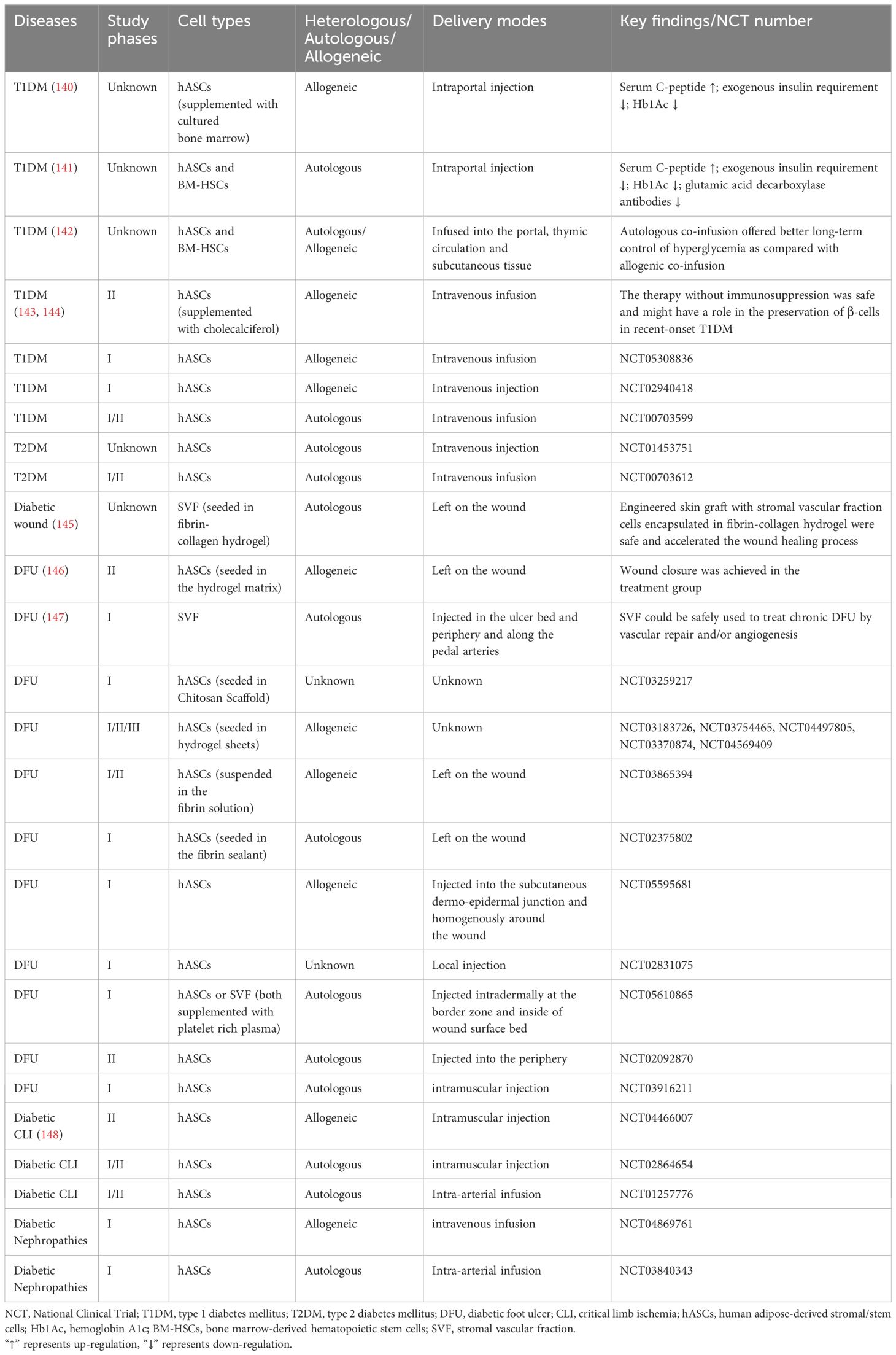
Table 2 Completed and ongoing clinical trials of ASCs in diabetes and its complications.
Unfortunately, their efficacy is primarily impeded by the limited expansion and survival of transplanted stem cells and their inability for proper functional integration in response to the physical environment ( 21 , 149 ). Moreover, only a fraction of MSCs successfully home to the pancreas and express insulin ( 150 ). 3D culture technology provides an opportunity to fill this knowledge gap. While there is a scarcity of research on the clinical application of 3D cultured ASCs, findings from animal and cellular studies suggest the potential benefits and advantages of 3D cultured ASCs in the treatment of diabetes ( Table 3 ).
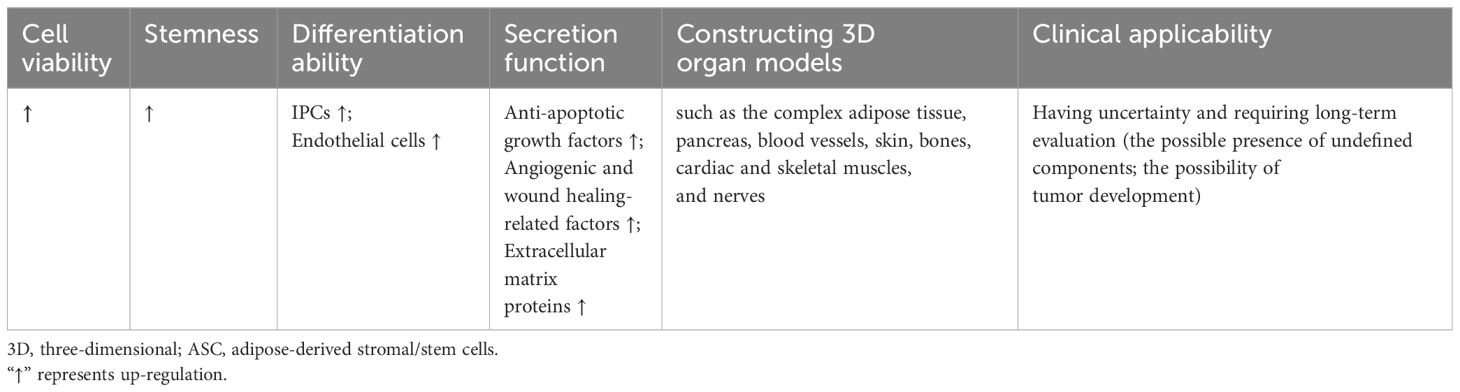
Table 3 The characteristics of 3D cultured ASCs in diabetes and diabetic complications compared to monolayer cells.
5.1 Promotion of insulin production
Type 2 diabetes mellitus (T2DM) is the most common type of diabetes, characterized by two interrelated metabolic defects: insulin resistance and pancreatic islet β-cell dysfunction. The development of T2DM is influenced by a complex interplay of genetic, environmental, emotional, and behavioral factors ( 151 ). Individuals with T2DM typically exhibit insulin resistance and gradual β-cell deterioration, resulting in insufficient insulin secretion, and consequent hyperglycemia and elevated free fatty acid levels. The resulting glucotoxicity and lipotoxicity exacerbate the dysfunction of β-cells to secrete insulin in response to hyperglycemia or oral hypoglycemic agents ( 16 ).
Type 1 diabetes mellitus (T1DM) is a chronic disease characterized by insulin deficiency resulting from autoimmune destruction of pancreatic islet β-cells, ultimately leading to hyperglycemia. Although the mechanism of T1DM in still not completely understood, it is believed to involve abnormalities in multiple immune cells, including T cells, B cells, regulatory T cells, monocytes and macrophages, and dendritic cells ( 152 ).
Given their similar outcome of pancreatic islet β-cell dysfunctions, the cell therapy as a potential strategy has attracted increased research attention. However, the transplantation of functional β-cells as a therapeutic strategy is impeded by the significant challenge of generating an adequate quantity of β-cells ex vivo and subsequently maintaining their viability post-transplantation. β-cells are susceptible to hypoxia and are prone to rapid apoptosis or damage as a result of the host immune response ( 153 ). Notably, the use of 3D cultured ASCs significantly promotes the construction and transplantation of islets and promote the insulin production.
Firstly, 3D cultured ASCs are capable to differentiate into insulin-producing cells (IPCs) to promote insulin production. For example, Khorsandi et al. found that the collagen/HA scaffold could enhance the differentiation of IPCs from rASCs. Compared to the 2D culture, the insulin release from 3D ASCs-derived IPCs showed up-regulation when exposed to a high glucose medium. The percentage of insulin-positive cells in 3D culture showed an approximately 4-fold increase compared to the 2D cultured cells ( 154 ). Ikemoto et al. developed a human recombinant peptide petaloid μ-piece 3D culture method to generate IPCs from hASCs. Following transplantation of 96 IPCs under the kidney capsule or intra-mesentery in STZ-induced diabetic nude mice, the hyperglycemic state was restored to normoglycemia ( 155 ). Ohta et al. found that blood glucose levels of STZ-induced diabetic nude mice were normalized after transplantation of 3D-cultured IPCs ( 156 ).
Secondly, the immunomodulatory action of 3D cultured ASCs can improve the micro-environment of islets. Abadpour et al. developed 3D-printed bioactive scaffolds containing islets and hASCs by combining alginate and nano-fibrillated cellulose bioink. Bioink diffusion properties were demonstrated, as well as benefits of hASCs for glucose sensing, insulin secretion, islet viability, and the reduction of pro-inflammatory cytokines, including growth-regulated protein-α and interferon gamma-induced protein-10 ( 157 ).
Furthermore, the 3D culture methods can also facilitate the survival of pancreatic islets and increase the functionality of grafts before transplantation. Jun et al. introduced a method of transplantation by co-culturing single primary islet cells with rASCs in concave microwells. These spheroids exhibited distinct ultrastructural morphologies, increased viability, and enhanced insulin secretion compared to mono-cultured islet spheroids, suggesting that ASCs may protect islet cells from damage by releasing anti-apoptotic growth factors. Additionally, the co-encapsulation of islets with additional ASCs within microfibers could further prolong graft survival through the anti-inflammatory properties of ASCs ( 22 ). Wang et al. effectively produced viable and functional heterocellular islet micro-tissues by combining islet cells, human umbilical vein endothelial cells, and hASCs within porcine decellularized ECM. These 3D islet micro-tissues exhibited sustained viability and normal secretory function, as well as heightened drug sensitivity during testing. Additionally, the utilization of 3D islet micro-tissues resulted in improved survival rates and enhanced graft function in murine models of diabetes ( 158 ).
In conclusion, 3D cultured ASC grafts play more significant role of insulin production through differentiating into IPCs, improving the micro-environment of islets, and enhancing survival and functionality.
5.2 Treatment of diabetic foot ulcer
Diabetic complications are mainly caused by high-glucose-induced cellular and molecular impairments and dysfunctions of cardiovascular and neural systems. While monolayer ASCs have demonstrated efficacy in treating a range of diabetic complications ( 139 ), the current studies about treatment of 3D ASCs are mainly focused on the DFU.
The diabetic foot ulcer, considered among the most severe types of diabetic wounds, has significant challenges to healing due to diabetic neuropathy, reduced blood flow, and infections ( 159 ). Non-healing ulcers may progress to gangrene, requiring foot amputations.
The normal wound healing process is characterized by four stages: hemostasis, inflammation, proliferation, and remodeling. In the hemostasis stage, vasoconstriction, platelet aggregation, and recruitment of circulating coagulation factors occur. The inflammation stage involves the gathering of inflammatory cells that secrete inflammatory factors like matrix metalloproteinase (MMP) and neutrophil extracellular traps (NETs). During the proliferation stage, the inflammation diminishes, and skin cells such as keratinocytes secrete EGF, proliferate, and migrate to the wound bed. During the process of tissue remodeling, new tissue is restructured and deposited via ECM and neovascularization, facilitated by fibroblasts secreting FGF and vascular endothelial cells secreting VEGF ( 160 – 162 ).
In diabetic wounds, tissue ischemia, hypoxia, and a high glucose microenvironment disrupt the normal progression of these healing stages, leading to delayed or non-healing of wounds and various clinical complications ( 163 ). Currently, DFUs are treated with vascular intervention therapy, drugs and other non-surgical therapies, such as dressing adjuvant therapy, hyperbaric oxygen therapy, hyperthermia and growth factor therapy ( 164 ). However, the efficacy of these approaches remains limited ( 159 ). Therefore, future research endeavors are anticipated to concentrate on more effective treatment strategies, with a particular emphasis on advancing stem cell-based therapies.
ASCs exhibit significant promise in the treatment of diabetic foot ulcers. Basically, the effects of ASCs rely on their promotion of immunomodulation, neovascularization and fibro synthesis ( 165 , 166 ). The routes of delivery of ASCs into the wound vary between direct injection (such as intradermal injection around the wound, intra-fascial, and intramuscular injection), topical gel treatment, engineered skin graft sheet, and with scaffolds. The survival rate and potency of expansion of ASCs in wound bed are limited in traditional injection. Therefore, scaffolds cell delivery systems are necessary which offer optimal environments for cell adhesion, proliferation, and differentiation ( 167 , 168 ).
A common solution involves seeding cells into hydrogels. Zeng et al. proposed that gelatin microcryogels (GMs) presented a novel method of cell delivery that could not only enhance wound bed healing but also directly influence the basal layer of the wound. They demonstrated that GMs provided an enhanced microenvironment for inducing endothelial cell differentiation of hASCs, thereby offering potential in vivo applications for angiogenic regeneration. Additionally, they demonstrated the priming effects of GMs on the upregulation of stemness genes and improved secretion of crucial growth factors in hASCs for wound healing, such as VEGF, HGF, basic fibroblast growth factor (bFGF), and platelet-derived growth factor BB (PDGFbb) ( 169 ). Feng et al. examined the therapeutic potential of hASCs cultured as micro-spheroids in the HA gel. Diabetic ulcers in mice with hASC spheroids resulted in accelerated wound epithelialization and increased dermal thickness, surpassing the outcomes observed with vehicle alone or monolayer-cultured ASCs ( 170 ). An injectable hydrogel system based on PEG and gelatin was examined for delivering hASCs into diabetic wounds. The stemness-linked transcription factor expression of hASCs was preserved in vitro and cell retention was significantly enhanced in vivo by this gel. In diabetic mice, this ASC-hydrogel treatment reduced inflammatory cell infiltration, enhanced neovascularization, and sped up wound closure ( 23 ).
There are also other bioengineering approaches for constructing 3D cultured ASCs. For example, Tyeb et al. introduced a combinatorial method involving the utilization of gelatin-sericin (GS) scaffolds coated with laminin (GSL). GS scaffolds provided enhanced protection against free radical-induced damage compared to gelatin scaffolds and consequently improved cell viability and metabolic function. The utilization of rASCs loaded onto GSL scaffolds resulted in enhanced regeneration, collagen remodeling, and increased expression of CD31 in diabetic ulcer rat models ( 171 ).
However, the broad use of matrix components aiding in the formation of 3D structures may impose constraints on the clinical applicability owing to the presence of undefined components. The implementation of hASCs formulated as multicellular aggregates without scaffolds also facilitated the healing wounds of diabetic mice. These aggregates exhibited a noteworthy increase in the production of extracellular matrix proteins including tenascin C, collagen VI α3, and fibronectin, as well as the secretion of soluble factors including HGF, MMP-2, and MMP-14 when compared to monolayer culture ( 172 ).
Considering that the main mechanism of cell action involves the paracrine effect, the characterization of components secreted by cells is vital, which indicates that ASCs can also function through their conditioned media. Lee et al. successfully fabricated an alginate-based scaffold using 3D printing and electrospinning techniques, which served as a structure to encapsulate hASC spheroids. This structure not only securely entrapped the spheroids but also facilitated the stable release of factors associated with angiogenesis and wound healing, such as CD31, VEGF, HGF, C-X-C chemokine receptor type 5 (CXCR5), IL-8, and MMP-1. They also demonstrated the role of these factors through a tube-forming assay and found that conditioned media from the spheroid-scaffold group enhanced the formation of capillary-like structures in human umbilical vein endothelial cells when compared to the single cell-scaffold group ( 173 ).
Utilizing diverse 3D culture techniques and materials such as hydrogels, bioactive scaffolds, scaffold-free methods, and conditioned media from 3D cultured cells, ASCs have the potential to facilitate diabetic wound healing by the promotion of immunomodulation, neovascularization and fibro synthesis.
5.3 Modelling tissues and organs
Aside from their application in diabetic therapy through transplantation, 3D cultured ASCs are crucial in the development of in vitro models that mimic the pathophysiology of different tissues and organs linked to diabetes and its associated complications. These models also potentially serve as valuable tools for screening novel therapeutic interventions and minimizing the reliance on animal experimentation.
Adipose tissue is a significant location of insulin resistance in individuals with type 2 diabetes mellitus (T2DM) and is linked to heightened chronic inflammation. The establishment of in vitro models for investigating the pathogenesis of adipose tissue in metabolic diseases would offer significant benefits. Numerous efforts have been made to create 3D adipose cultures utilizing ASCs. For instance, hASCs were cultivated on plates coated with ELP–PEI copolymer, as the PEI component promotes spheroid formation and the ELP component facilitates the attachment of spheroids to the surface. This culture platform enabled the production of functional adipocytes that exhibited a favorable response to fatty acid stimulation ( 126 ). Moreover, Gerlach et al. utilized multicompartment hollow fiber-based bioreactor technology to generate 3D adipose tissue. In vitro, 3D bioreactors allowed greater metabolic activity compared with traditional 2D cultured hASCs and enabled the generation of adipose tissue as long as two months ( 174 ). Yang et al. created a 3D human adipose microtissue engineered within a microfluidic system ( 175 ). Furthermore, culture technologies have been employed in the generation of beige or brown adipose tissue ( 176 – 178 ). As the characterization of ASCs can be influenced by the source of adipose tissue, the availability of such tools presents a wide range of opportunities in vitro studies. By utilizing these models, it becomes feasible to compare relative metabolic responses of adipose depots under different health conditions to metabolic researches.
An ideal and comprehensive adipose tissue models should include all in vivo components, such as adipocytes, connective tissues, veins and nerves. For example, Lau et al. described an adipose micro-physiological system that involved sandwiching human WAT between tissue-engineered sheets of ASCs. The use of ASCs provided a structural ECM framework to encompass and support the mature adipocytes as well as paracrine growth factors ( 179 ). One common approach in the generation of vascularized adipose tissue involves the inclusion of exogenous endothelial cells through co-culture ( 180 – 182 ). The utilization of vascularized adipose models presents a promising avenue for developing novel drugs to treat metabolic diseases by modulation of the adipose vasculature. Moreover, adipose depots could be infiltrated with inflammatory and immune cells during preparation or after differentiation into adipocytes ( 183 ), offering a valuable tool for immune–metabolic research.
Furthermore, through differentiation and secretory capabilities of ASCs, it becomes possible to connect them with micro-physiological systems representing other organs. Despite being in the early stages of development, 3D models simulating organs such as the pancreas, blood vessels, skin, bones, cardiac and skeletal muscles, and nerves ( Table 4 ), exhibit promising potential in mimicking the effects of diabetes and its complications, as well as evaluating the efficacy of cell transplantation therapy.
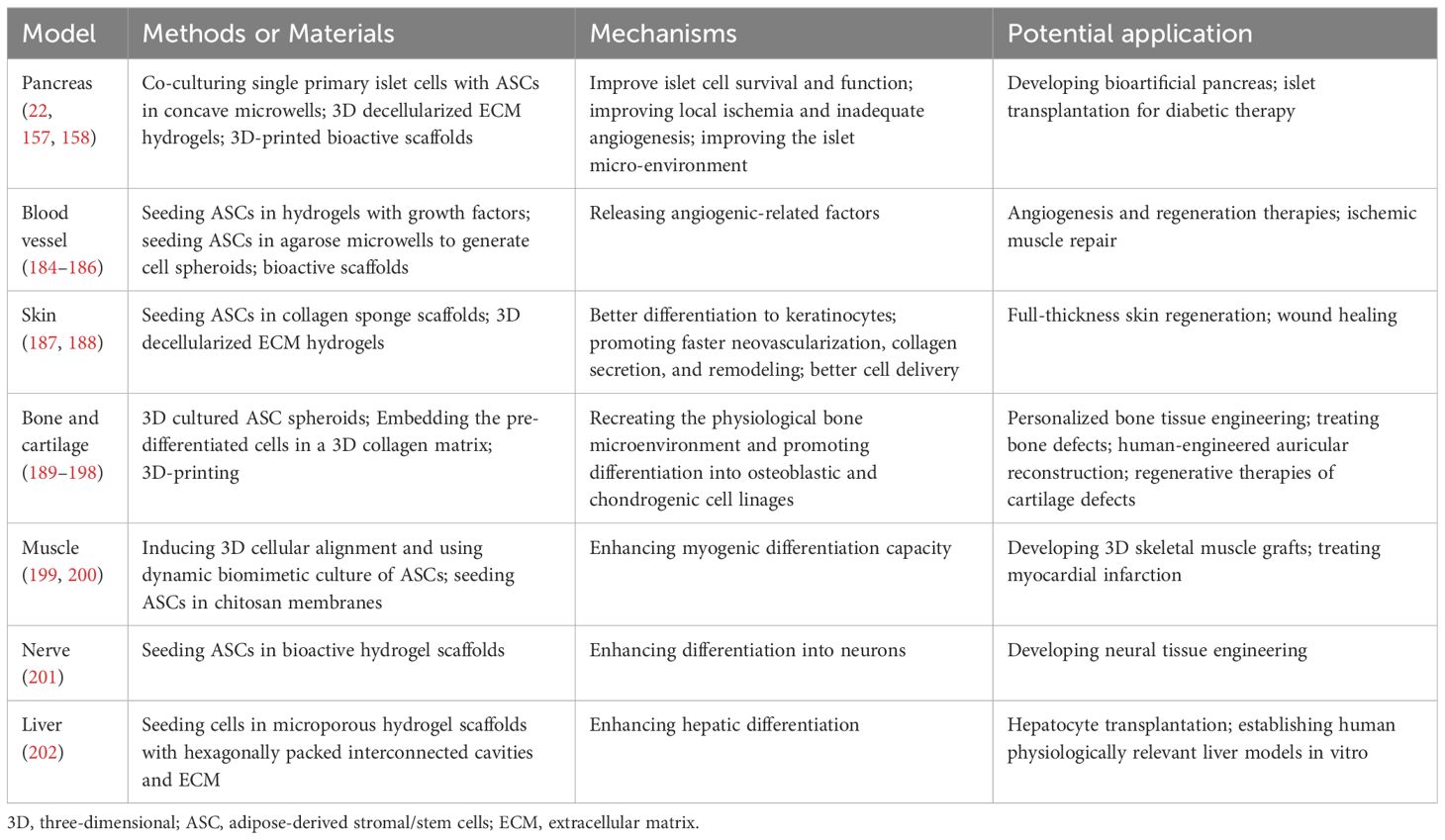
Table 4 Examples about 3D cultured ASC models of various organs except adipose tissues.
6 Conclusions and future prospects
3D cultured cells have been advantageous in various biomedical fields. This technology is still in the early stages. The isolation, culture, and identification of cells are the basis of 3D culture. Therefore, large-scale manufacturing methods incorporating quality control are necessary for producing cells and 3D cultured transplantations.
Indeed, 2D adherent cell culture of ASCs is still conventionally used for both in vitro and in vivo studies. These cells have been extensively characterized, whereas many factors have not been analyzed on 3D cultured ASCs yet. Additionally, while monolayer ASC cells have shown effects for the treatment of diabetes and its complications in both clinical trials and animal experiments, current research status on 3D cultured ASCs mainly concentrates on T1DM and DFU only. Thus, further research is required to better understand the function and underlying mechanisms of 3D cultured ASC therapy.
The potential side effects of ASCs for tumor development should not be disregarded in studies; however, they may also serve as a potential tool for antitumor therapies. While tumor cells altering the phenotype and function of in vitro cultured ASCs through paracrine mechanisms ( 203 ), ASCs can also serve as a factor that promotes tumor growth ( 203 – 205 ). By contrast, ASC exosomes were shown to possess immunomodulatory properties and can inhibit cancer growth, migration, and colony formation ( 206 ). A strategy for tumor therapy used ASCs which loaded gold nanorod (AuNR)-PEG-poly(ethyleneimine) (APP) and Chlorin e6 (Ce6). Following activation of the APP/Ce6 agents through irradiation, ASCs were shown to play a role in tumor migration, tropism, and exhibit anticancer properties ( 207 ). These findings underscore the importance of exercising caution in the utilization of 3D cultured ASCs, with long-term experiments necessary to assess their safety. Specifically, careful consideration should be given to the potential of 3D culture to induce tumorigenesis while enhancing cell viability and stemness.
Author contributions
YS: Conceptualization, Writing – original draft. XY: Conceptualization, Writing – original draft. JM: Writing – review & editing. WK: Writing – review & editing. XH: Writing – review & editing. JZ: Writing – review & editing. LC: Project administration, Supervision, Writing – review & editing.
The author(s) declare financial support was received for the research, authorship, and/or publication of this article. This research was supported by the National Natural Science Foundation of China (82170822, 82070809, 82300895, and 81900734).
Acknowledgments
The authors thank Figdraw for providing access to create figures.
Conflict of interest
The authors declare that the research was conducted in the absence of any commercial or financial relationships that could be construed as a potential conflict of interest.
Publisher’s note
All claims expressed in this article are solely those of the authors and do not necessarily represent those of their affiliated organizations, or those of the publisher, the editors and the reviewers. Any product that may be evaluated in this article, or claim that may be made by its manufacturer, is not guaranteed or endorsed by the publisher.
1. Hoang DM, Pham PT, Bach TQ, Ngo ATL, Nguyen QT, Phan TTK, et al. Stem cell-based therapy for human diseases. Signal Transduct Target Ther . (2022) 7:272. doi: 10.1038/s41392-022-01134-4
PubMed Abstract | CrossRef Full Text | Google Scholar
2. Gepstein L. Derivation and potential applications of human embryonic stem cells. Circ Res . (2002) 91:866–76. doi: 10.1161/01.res.0000041435.95082.84
3. Cyranoski D. How human embryonic stem cells sparked a revolution. Nature . (2018) 555:428–30. doi: 10.1038/d41586-018-03268-4
4. Ho J, Yue D, Cheema U, Hsia HC, Dardik A. Innovations in stem cell therapy for diabetic wound healing. Adv Wound Care (New Rochelle) . (2023) 12:626–43. doi: 10.1089/wound.2021.0104
5. Yu J, Vodyanik MA, Smuga-Otto K, Antosiewicz-Bourget J, Frane JL, Tian S, et al. Induced pluripotent stem cell lines derived from human somatic cells. Science . (2007) 318:1917–20. doi: 10.1126/science.1151526
6. Yu J, Hu K, Smuga-Otto K, Tian S, Stewart R, Slukvin II, et al. Human induced pluripotent stem cells free of vector and transgene sequences. Science . (2009) 324:797–801. doi: 10.1126/science.1172482
7. Zhao T, Zhang ZN, Rong Z, Xu Y. Immunogenicity of induced pluripotent stem cells. Nature . (2011) 474:212–5. doi: 10.1038/nature10135
8. Lee AS, Tang C, Rao MS, Weissman IL, Wu JC. Tumorigenicity as a clinical hurdle for pluripotent stem cell therapies. Nat Med . (2013) 19:998–1004. doi: 10.1038/nm.3267
9. Viswanathan S, Shi Y, Galipeau J, Krampera M, Leblanc K, Martin I, et al. Mesenchymal stem versus stromal cells: International society for cell & Gene therapy (Isct®) mesenchymal stromal cell committee position statement on nomenclature. Cytotherapy . (2019) 21:1019–24. doi: 10.1016/j.jcyt.2019.08.002
10. Bourin P, Bunnell BA, Casteilla L, Dominici M, Katz AJ, March KL, et al. Stromal cells from the adipose tissue-derived stromal vascular fraction and culture expanded adipose tissue-derived stromal/stem cells: A joint statement of the international federation for adipose therapeutics and science (Ifats) and the international society for cellular therapy (Isct). Cytotherapy . (2013) 15:641–8. doi: 10.1016/j.jcyt.2013.02.006
11. Qi Y, Ma J, Li S, Liu W. Applicability of adipose-derived mesenchymal stem cells in treatment of patients with type 2 diabetes. Stem Cell Res Ther . (2019) 10:274. doi: 10.1186/s13287-019-1362-2
12. Mundra V, Gerling IC, Mahato RI. Mesenchymal stem cell-based therapy. Mol Pharm . (2013) 10:77–89. doi: 10.1021/mp3005148
13. Bacakova L, Zarubova J, Travnickova M, Musilkova J, Pajorova J, Slepicka P, et al. Stem cells: Their source, potency and use in regenerative therapies with focus on adipose-derived stem cells - a review. Biotechnol Adv . (2018) 36:1111–26. doi: 10.1016/j.bioteChadv.2018.03.011
14. Lin PC, Chiou TW, Lin ZS, Huang KC, Lin YC, Huang PC, et al. A proposed novel stem cell therapy protocol for liver cirrhosis. Cell Transplant . (2015) 24:533–40. doi: 10.3727/096368915x687228
15. Ong KL, Stafford LK, McLaughlin SA, Boyko EJ, Vollset SE. Global, regional, and national burden of diabetes from 1990 to 2021, with projections of prevalence to 2050: A systematic analysis for the global burden of disease study 2021. Lancet . (2023) 402:203–34. doi: 10.1016/s0140-6736(23)01301-6
16. Nyenwe EA, Jerkins TW, Umpierrez GE, Kitabchi AE. Management of type 2 diabetes: Evolving strategies for the treatment of patients with type 2 diabetes. Metabolism . (2011) 60:1–23. doi: 10.1016/j.metabol.2010.09.010
17. Tan SY, Mei Wong JL, Sim YJ, Wong SS, Mohamed Elhassan SA, Tan SH, et al. Type 1 and 2 diabetes mellitus: A review on current treatment approach and gene therapy as potential intervention. Diabetes Metab Syndr . (2019) 13:364–72. doi: 10.1016/j.dsx.2018.10.008
18. Lorberbaum DS, Sarbaugh D, Sussel L. Leveraging the strengths of mice, human stem cells, and organoids to model pancreas development and diabetes. Front Endocrinol (Lausanne) . (2022) 13:1042611. doi: 10.3389/fendo.2022.1042611
19. Niclauss N, Morel P, Berney T. Has the gap between pancreas and islet transplantation closed? Transplantation . (2014) 98:593–9. doi: 10.1097/tp.0000000000000288
20. Hu C, Jia W. Therapeutic medications against diabetes: What we have and what we expect. Adv Drug Delivery Rev . (2019) 139:3–15. doi: 10.1016/j.addr.2018.11.008
CrossRef Full Text | Google Scholar
21. Lin HP, Chan TM, Fu RH, Chuu CP, Chiu SC, Tseng YH, et al. Applicability of adipose-derived stem cells in type 1 diabetes mellitus. Cell Transplant . (2015) 24:521–32. doi: 10.3727/096368915x686977
22. Jun Y, Kang AR, Lee JS, Park SJ, Lee DY, Moon SH, et al. Microchip-based engineering of super-pancreatic islets supported by adipose-derived stem cells. Biomaterials . (2014) 35:4815–26. doi: 10.1016/j.biomaterials.2014.02.045
23. Dong Y, Rodrigues M, Kwon SH, Li X, Sigen A, Brett EA, et al. Acceleration of diabetic wound regeneration using an in situ-formed stem-cell-based skin substitute. Adv Healthc Mater . (2018) 7:e1800432. doi: 10.1002/adhm.201800432
24. Cai L, Dewi RE, Heilshorn SC. Injectable hydrogels with in situ double network formation enhance retention of transplanted stem cells. Adv Funct Mater . (2015) 25:1344–51. doi: 10.1002/adfm.201403631
25. Aguado BA, Mulyasasmita W, Su J, Lampe KJ, Heilshorn SC. Improving viability of stem cells during syringe needle flow through the design of hydrogel cell carriers. Tissue Eng Part A . (2012) 18:806–15. doi: 10.1089/ten.TEA.2011.0391
26. Di Stefano AB, Urrata V, Trapani M, Moschella F, Cordova A, Toia F. Systematic review on spheroids from adipose-derived stem cells: Spontaneous or artefact state? J Cell Physiol . (2022) 237:4397–411. doi: 10.1002/jcp.30892
27. Baptista LS, Silva KR, Jobeili L, Guillot L, Sigaudo-Roussel D. Unraveling white adipose tissue heterogeneity and obesity by adipose stem/stromal cell biology and 3d culture models. Cells . (2023) 12:1583. doi: 10.3390/cells12121583
28. Abbott A. Cell culture: Biology's new dimension. Nature . (2003) 424:870–2. doi: 10.1038/424870a
29. Hu W, Lazar MA. Modelling metabolic diseases and drug response using stem cells and organoids. Nat Rev Endocrinol . (2022) 18:744–59. doi: 10.1038/s41574-022-00733-z
30. Sipp D, Robey PG, Turner L. Clear up this stem-cell mess. Nature . (2018) 561:455–7. doi: 10.1038/d41586-018-06756-9
31. Dominici M, Le Blanc K, Mueller I, Slaper-Cortenbach I, Marini F, Krause D, et al. Minimal criteria for defining multipotent mesenchymal stromal cells. The international society for cellular therapy position statement. Cytotherapy . (2006) 8:315–7. doi: 10.1080/14653240600855905
32. Viswanathan S, Ciccocioppo R, Galipeau J, Krampera M, Le Blanc K, Martin I, et al. Consensus international council for commonality in blood banking automation-international society for cell & Gene therapy statement on standard nomenclature abbreviations for the tissue of origin of mesenchymal stromal cells. Cytotherapy . (2021) 23:1060–3. doi: 10.1016/j.jcyt.2021.04.009
33. Caplan AI. Mesenchymal stem cells: Time to change the name! Stem Cells Transl Med . (2017) 6:1445–51. doi: 10.1002/sctm.17-0051
34. Renesme L, Pierro M, Cobey KD, Mital R, Nangle K, Shorr R, et al. Definition and characteristics of mesenchymal stromal cells in preclinical and clinical studies: A scoping review. Stem Cells Transl Med . (2022) 11:44–54. doi: 10.1093/stcltm/szab009
35. Rodbell M. Metabolism of Isolated Fat Cells. Ii. The Similar Effects of Phospholipase C (Clostridium perfringens alpha toxin) and of insulin on glucose and amino acid metabolism. J Biol Chem . (1966) 241:130–9. doi: 10.1016/S0021-9258(18)96967-X
36. Rodbell M, Jones AB. Metabolism of isolated fat cells. 3. The similar inhibitory action of phospholipase C (Clostridium perfringens alpha toxin) and of insulin on lipolysis stimulated by lipolytic hormones and theophylline. J Biol Chem . (1966) 241:140–2. doi: 10.1016/S0021-9258(18)96968-1
37. Rodbell M. The metabolism of isolated fat cells. Iv. Regulation of release of protein by lipolytic hormones and insulin. J Biol Chem . (1966) 241:3909–17. doi: 10.1016/S0021-9258(18)99793-0
38. Zuk PA, Zhu M, Mizuno H, Huang J, Futrell JW, Katz AJ, et al. Multilineage cells from human adipose tissue: Implications for Cell-Based Therapies. Tissue Eng . (2001) 7:211–28. doi: 10.1089/107632701300062859
39. Zhu Y, Liu T, Song K, Fan X, Ma X, Cui Z. Adipose-Derived Stem Cell: A Better Stem Cell Than Bmsc. Cell Biochem Funct . (2008) 26:664–75. doi: 10.1002/cbf.1488
40. Al-Ghadban S, Bunnell BA. Adipose Tissue-Derived Stem Cells: Immunomodulatory effects and therapeutic potential. Physiol (Bethesda) . (2020) 35:125–33. doi: 10.1152/physiol.00021.2019
41. Gimble JM, Katz AJ, Bunnell BA. Adipose-derived stem cells for regenerative medicine. Circ Res . (2007) 100:1249–60. doi: 10.1161/01.Res.0000265074.83288.09
42. Rennert RC, Sorkin M, Januszyk M, Duscher D, Kosaraju R, Chung MT, et al. Diabetes impairs the angiogenic potential of adipose-derived stem cells by selectively depleting cellular subpopulations. Stem Cell Res Ther . (2014) 5:79. doi: 10.1186/scrt468
43. Cianfarani F, Toietta G, Di Rocco G, Cesareo E, Zambruno G, Odorisio T. Diabetes impairs adipose tissue-derived stem cell function and efficiency in promoting wound healing. Wound Repair Regener . (2013) 21:545–53. doi: 10.1111/wrr.12051
44. El-Ftesi S, Chang EI, Longaker MT, Gurtner GC. Aging and diabetes impair the neovascular potential of adipose-derived stromal cells. Plast Reconstr Surg . (2009) 123:475–85. doi: 10.1097/PRS.0b013e3181954d08
45. Wang M, Song L, Strange C, Dong X, Wang H. Therapeutic effects of adipose stem cells from diabetic mice for the treatment of type 2 diabetes. Mol Ther . (2018) 26:1921–30. doi: 10.1016/j.ymthe.2018.06.013
46. An R, Zhang Y, Qiao Y, Song L, Wang H, Dong X. Adipose stem cells isolated from diabetic mice improve cutaneous wound healing in streptozotocin-induced diabetic mice. Stem Cell Res Ther . (2020) 11:120. doi: 10.1186/s13287-020-01621-x
47. Uzun E, Güney A, Gönen ZB, Özkul Y, Kafadar İH, Günay M, et al. Intralesional allogeneic adipose-derived stem cells application in chronic diabetic foot ulcer: Phase I/2 safety study. Foot Ankle Surg . (2021) 27:636–42. doi: 10.1016/j.fas.2020.08.002
48. Deptuła M, Brzezicka A, Skoniecka A, Zieliński J, Pikuła M. Adipose-derived stromal cells for nonhealing wounds: Emerging opportunities and challenges. Med Res Rev . (2021) 41:2130–71. doi: 10.1002/med.21789
49. Louwen F, Ritter A, Kreis NN, Yuan J. Insight into the development of obesity: Functional alterations of adipose-derived mesenchymal stem cells. Obes Rev . (2018) 19:888–904. doi: 10.1111/obr.12679
50. García-Contreras M, Vera-Donoso CD, Hernández-Andreu JM, García-Verdugo JM, Oltra E. Therapeutic potential of human adipose-derived stem cells (Adscs) from cancer patients: A pilot study. PLoS One . (2014) 9:e113288. doi: 10.1371/journal.pone.0113288
51. Mieczkowska A, Schumacher A, Filipowicz N, Wardowska A, Zieliński M, Madanecki P, et al. Immunophenotyping and transcriptional profiling of in vitro cultured human adipose tissue derived stem cells. Sci Rep . (2018) 8:11339. doi: 10.1038/s41598-018-29477-5
52. Bunnell BA. Adipose tissue-derived mesenchymal stem cells. Cells . (2021) 10:3433. doi: 10.3390/cells10123433
53. Ritter A, Friemel A, Roth S, Kreis NN, Hoock SC, Safdar BK, et al. Subcutaneous and visceral adipose-derived mesenchymal stem cells: Commonality and diversity. Cells . (2019) 8:1288. doi: 10.3390/cells8101288
54. Macotela Y, Emanuelli B, Mori MA, Gesta S, Schulz TJ, Tseng YH, et al. Intrinsic differences in adipocyte precursor cells from different white fat depots. Diabetes . (2012) 61:1691–9. doi: 10.2337/db11-1753
55. Tang Y, Pan ZY, Zou Y, He Y, Yang PY, Tang QQ, et al. A comparative assessment of adipose-derived stem cells from subcutaneous and visceral fat as a potential cell source for knee osteoarthritis treatment. J Cell Mol Med . (2017) 21:2153–62. doi: 10.1111/jcmm.13138
56. Wada Y, Ikemoto T, Morine Y, Imura S, Saito Y, Yamada S, et al. The differences in the characteristics of insulin-producing cells using human adipose-tissue derived mesenchymal stem cells from subcutaneous and visceral tissues. Sci Rep . (2019) 9:13204. doi: 10.1038/s41598-019-49701-0
57. Silva FJ, Holt DJ, Vargas V, Yockman J, Boudina S, Atkinson D, et al. Metabolically active human brown adipose tissue derived stem cells. Stem Cells . (2014) 32:572–81. doi: 10.1002/stem.1595
58. Di Franco A, Guasti D, Squecco R, Mazzanti B, Rossi F, Idrizaj E, et al. Searching for classical brown fat in humans: Development of a novel human fetal brown stem cell model. Stem Cells . (2016) 34:1679–91. doi: 10.1002/stem.2336
59. Carvalho PP, Gimble JM, Dias IR, Gomes ME, Reis RL. Xenofree enzymatic products for the isolation of human adipose-derived stromal/stem cells. Tissue Eng Part C Methods . (2013) 19:473–8. doi: 10.1089/ten.TEC.2012.0465
60. Kølle SF, Fischer-Nielsen A, Mathiasen AB, Elberg JJ, Oliveri RS, Glovinski PV, et al. Enrichment of autologous fat grafts with ex-vivo expanded adipose tissue-derived stem cells for graft survival: A randomised placebo-controlled trial. Lancet . (2013) 382:1113–20. doi: 10.1016/s0140-6736(13)61410-5
61. Follin B, Tratwal J, Haack-Sørensen M, Elberg JJ, Kastrup J, Ekblond A. Identical effects of vegf and serum-deprivation on phenotype and function of adipose-derived stromal cells from healthy donors and patients with ischemic heart disease. J Transl Med . (2013) 11:219. doi: 10.1186/1479-5876-11-219
62. Lee MS, Youn C, Kim JH, Park BJ, Ahn J, Hong S, et al. Enhanced cell growth of adipocyte-derived mesenchymal stem cells using chemically-defined serum-free media. Int J Mol Sci . (2017) 18:1779. doi: 10.3390/ijms18081779
63. Dessels C, Ambele MA, Pepper MS. The effect of medium supplementation and serial passaging on the transcriptome of human adipose-derived stromal cells expanded in vitro. Stem Cell Res Ther . (2019) 10:253. doi: 10.1186/s13287-019-1370-2
64. Riis S, Nielsen FM, Pennisi CP, Zachar V, Fink T. Comparative analysis of media and supplements on initiation and expansion of adipose-derived stem cells. Stem Cells Transl Med . (2016) 5:314–24. doi: 10.5966/sctm.2015-0148
65. Kocaoemer A, Kern S, Klüter H, Bieback K. Human ab serum and thrombin-activated platelet-rich plasma are suitable alternatives to fetal calf serum for the expansion of mesenchymal stem cells from adipose tissue. Stem Cells . (2007) 25:1270–8. doi: 10.1634/stemcells.2006-0627
66. Bieback K, Ha VA, Hecker A, Grassl M, Kinzebach S, Solz H, et al. Altered gene expression in human adipose stem cells cultured with fetal bovine serum compared to human supplements. Tissue Eng Part A . (2010) 16:3467–84. doi: 10.1089/ten.TEA.2009.0727
67. Tirza G, Solodeev I, Sela M, Greenberg I, Pasmanik-Chor M, Gur E, et al. Reduced culture temperature attenuates oxidative stress and inflammatory response facilitating expansion and differentiation of adipose-derived stem cells. Stem Cell Res Ther . (2020) 11:35. doi: 10.1186/s13287-019-1542-0
68. Mohyeldin A, Garzón-Muvdi T, Quiñones-Hinojosa A. Oxygen in stem cell biology: A critical component of the stem cell niche. Cell Stem Cell . (2010) 7:150–61. doi: 10.1016/j.stem.2010.07.007
69. Lee EY, Xia Y, Kim WS, Kim MH, Kim TH, Kim KJ, et al. Hypoxia-enhanced wound-healing function of adipose-derived stem cells: Increase in stem cell proliferation and up-regulation of vegf and bfgf. Wound Repair Regener . (2009) 17:540–7. doi: 10.1111/j.1524-475X.2009.00499.x
70. Zanata F, Bowles A, Frazier T, Curley JL, Bunnell BA, Wu X, et al. Effect of cryopreservation on human adipose tissue and isolated stromal vascular fraction cells: In vitro and in vivo analyses. Plast Reconstr Surg . (2018) 141:232e–43e. doi: 10.1097/prs.0000000000004030
71. Devitt SM, Carter CM, Dierov R, Weiss S, Gersch RP, Percec I. Successful isolation of viable adipose-derived stem cells from human adipose tissue subject to long-term cryopreservation: positive implications for adult stem cell-based therapeutics in patients of advanced age. Stem Cells Int . (2015) 2015:146421. doi: 10.1155/2015/146421
72. Park S, Lee DR, Nam JS, Ahn CW, Kim H. Fetal bovine serum-free cryopreservation methods for clinical banking of human adipose-derived stem cells. Cryobiology . (2018) 81:65–73. doi: 10.1016/j.cryobiol.2018.02.008
73. Søndergaard RH, Follin B, Lund LD, Juhl M, Ekblond A, Kastrup J, et al. Senescence and quiescence in adipose-derived stromal cells: Effects of human platelet lysate, fetal bovine serum and hypoxia. Cytotherapy . (2017) 19:95–106. doi: 10.1016/j.jcyt.2016.09.006
74. Neri S, Bourin P, Peyrafitte JA, Cattini L, Facchini A, Mariani E. Human adipose stromal cells (Asc) for the regeneration of injured cartilage display genetic stability after in vitro culture expansion. PLoS One . (2013) 8:e77895. doi: 10.1371/journal.pone.0077895
75. Yin Q, Xu N, Xu D, Dong M, Shi X, Wang Y, et al. Comparison of senescence-related changes between three- and two-dimensional cultured adipose-derived mesenchymal stem cells. Stem Cell Res Ther . (2020) 11:226. doi: 10.1186/s13287-020-01744-1
76. Estrada JC, Torres Y, Benguría A, Dopazo A, Roche E, Carrera-Quintanar L, et al. Human mesenchymal stem cell-replicative senescence and oxidative stress are closely linked to aneuploidy. Cell Death Dis . (2013) 4:e691. doi: 10.1038/cddis.2013.211
77. Efimenko AY, Kochegura TN, Akopyan ZA, Parfyonova YV. Autologous stem cell therapy: How aging and chronic diseases affect stem and progenitor cells. Biores Open Access . (2015) 4:26–38. doi: 10.1089/biores.2014.0042
78. Fridman AL, Tainsky MA. Critical pathways in cellular senescence and immortalization revealed by gene expression profiling. Oncogene . (2008) 27:5975–87. doi: 10.1038/onc.2008.213
79. Kang SK, Putnam L, Dufour J, Ylostalo J, Jung JS, Bunnell BA. Expression of telomerase extends the lifespan and enhances osteogenic differentiation of adipose tissue-derived stromal cells. Stem Cells . (2004) 22:1356–72. doi: 10.1634/stemcells.2004-0023
80. Tchkonia T, Giorgadze N, Pirtskhalava T, Thomou T, DePonte M, Koo A, et al. Fat depot-specific characteristics are retained in strains derived from single human preadipocytes. Diabetes . (2006) 55:2571–8. doi: 10.2337/db06-0540
81. Wolbank S, Stadler G, Peterbauer A, Gillich A, Karbiener M, Streubel B, et al. Telomerase immortalized human amnion- and adipose-derived mesenchymal stem cells: maintenance of differentiation and immunomodulatory characteristics. Tissue Eng Part A . (2009) 15:1843–54. doi: 10.1089/ten.tea.2008.0205
82. Shamsi F, Tseng YH. Protocols for generation of immortalized human brown and white preadipocyte cell lines. Methods Mol Biol . (2017) 1566:77–85. doi: 10.1007/978-1-4939-6820-6_8
83. Comas F, Latorre J, Ortega F, Oliveras-Cañellas N, Lluch A, Ricart W, et al. Permanent cystathionine-Β-synthase gene knockdown promotes inflammation and oxidative stress in immortalized human adipose-derived mesenchymal stem cells, enhancing their adipogenic capacity. Redox Biol . (2021) 42:101668. doi: 10.1016/j.redox.2020.101668
84. Huang M, Claussnitzer M, Saadat A, Coral DE, Kalamajski S, Franks PW. Engineered allele substitution at ppargc1a rs8192678 alters human white adipocyte differentiation, lipogenesis, and pgc-1α Content and turnover. Diabetologia . (2023) 66:1289–305. doi: 10.1007/s00125-023-05915-6
85. Iacomi DM, Rosca AM, Tutuianu R, Neagu TP, Pruna V, Simionescu M, et al. Generation of an immortalized human adipose-derived mesenchymal stromal cell line suitable for wound healing therapy. Int J Mol Sci . (2022) 23:8925. doi: 10.3390/ijms23168925
86. Tátrai P, Szepesi Á, Matula Z, Szigeti A, Buchan G, Mádi A, et al. Combined introduction of bmi-1 and htert immortalizes human adipose tissue-derived stromal cells with low risk of transformation. Biochem Biophys Res Commun . (2012) 422:28–35. doi: 10.1016/j.bbrc.2012.04.088
87. Balducci L, Blasi A, Saldarelli M, Soleti A, Pessina A, Bonomi A, et al. Immortalization of human adipose-derived stromal cells: Production of cell lines with high growth rate, mesenchymal marker expression and capability to secrete high levels of angiogenic factors. Stem Cell Res Ther . (2014) 5:63. doi: 10.1186/scrt452
88. Darimont C, Zbinden I, Avanti O, Leone-Vautravers P, Giusti V, Burckhardt P, et al. Reconstitution of telomerase activity combined with hpv-E7 expression allow human preadipocytes to preserve their differentiation capacity after immortalization. Cell Death Differ . (2003) 10:1025–31. doi: 10.1038/sj.cdd.4401273
89. Cherington V, Brown M, Paucha E, St Louis J, Spiegelman BM, Roberts TM. Separation of simian virus 40 large-T-antigen-transforming and origin-binding functions from the ability to block differentiation. Mol Cell Biol . (1988) 8:1380–4. doi: 10.1128/mcb.8.3.1380-1384.1988
90. Kretzschmar K, Clevers H. Organoids: modeling development and the stem cell niche in a dish. Dev Cell . (2016) 38:590–600. doi: 10.1016/j.devcel.2016.08.014
91. Kaushik G, Ponnusamy MP, Batra SK. Concise review: Current status of three-dimensional organoids as preclinical models. Stem Cells . (2018) 36:1329–40. doi: 10.1002/stem.2852
92. Zhou T, Tan L, Cederquist GY, Fan Y, Hartley BJ, Mukherjee S, et al. High-content screening in hpsc-neural progenitors identifies drug candidates that inhibit zika virus infection in fetal-like organoids and adult brain. Cell Stem Cell . (2017) 21:274–83.e5. doi: 10.1016/j.stem.2017.06.017
93. Camp JG, Badsha F, Florio M, Kanton S, Gerber T, Wilsch-Bräuninger M, et al. Human cerebral organoids recapitulate gene expression programs of fetal neocortex development. Proc Natl Acad Sci U.S.A . (2015) 112:15672–7. doi: 10.1073/pnas.1520760112
94. Pain B. Organoids in domestic animals: With which stem cells? Vet Res . (2021) 52:38. doi: 10.1186/s13567-021-00911-3
95. Ramalho-Santos M, Yoon S, Matsuzaki Y, Mulligan RC, Melton DA. "Stemness": transcriptional profiling of embryonic and adult stem cells. Science . (2002) 298:597–600. doi: 10.1126/science.1072530
96. Watt FM, Hogan BL. Out of eden: stem cells and their niches. Science . (2000) 287:1427–30. doi: 10.1126/science.287.5457.1427
97. Yan XZ, van den Beucken JJ, Both SK, Yang PS, Jansen JA, Yang F. Biomaterial strategies for stem cell maintenance during in vitro expansion. Tissue Eng Part B Rev . (2014) 20:340–54. doi: 10.1089/ten.TEB.2013.0349
98. Marx V. Cell culture: A better brew. Nature . (2013) 496:253–8. doi: 10.1038/496253a
99. Gibler P, Gimble J, Hamel K, Rogers E, Henderson M, Wu X, et al. Human adipose-derived stromal/stem cell culture and analysis methods for adipose tissue modeling in vitro: A systematic review. Cells . (2021) 10:1378. doi: 10.3390/cells10061378
100. Guo X, Li S, Ji Q, Lian R, Chen J. Enhanced viability and neural differential potential in poor post-thaw hadscs by agarose multi-well dishes and spheroid culture. Hum Cell . (2015) 28:175–89. doi: 10.1007/s13577-015-0116-4
101. Coyle R, Yao J, Richards D, Mei Y. The effects of metabolic substrate availability on human adipose-derived stem cell spheroid survival. Tissue Eng Part A . (2019) 25:620–31. doi: 10.1089/ten.TEA.2018.0163
102. Di Stefano AB, Grisafi F, Perez-Alea M, Castiglia M, Di Simone M, Meraviglia S, et al. Cell quality evaluation with gene expression analysis of spheroids (3d) and adherent (2d) adipose stem cells. Gene . (2021) 768:145269. doi: 10.1016/j.gene.2020.145269
103. Rybkowska P, Radoszkiewicz K, Kawalec M, Dymkowska D, Zabłocka B, Zabłocki K, et al. The metabolic changes between monolayer (2d) and three-dimensional (3d) culture conditions in human mesenchymal stem/stromal cells derived from adipose tissue. Cells . (2023) 12:178. doi: 10.3390/cells12010178
104. Yipeng J, Yongde X, Yuanyi W, Jilei S, Jiaxiang G, Jiangping G, et al. Microtissues enhance smooth muscle differentiation and cell viability of hadscs for three dimensional bioprinting. Front Physiol . (2017) 8:534. doi: 10.3389/fphys.2017.00534
105. Bhang SH, Cho SW, La WG, Lee TJ, Yang HS, Sun AY, et al. Angiogenesis in ischemic tissue produced by spheroid grafting of human adipose-derived stromal cells. Biomaterials . (2011) 32:2734–47. doi: 10.1016/j.biomaterials.2010.12.035
106. Al-Ghadban S, Artiles M, Bunnell BA. Adipose stem cells in regenerative medicine: looking forward. Front Bioeng Biotechnol . (2021) 9:837464. doi: 10.3389/fbioe.2021.837464
107. Hsu SH, Ho TT, Huang NC, Yao CL, Peng LH, Dai NT. Substrate-dependent modulation of 3d spheroid morphology self-assembled in mesenchymal stem cell-endothelial progenitor cell coculture. Biomaterials . (2014) 35:7295–307. doi: 10.1016/j.biomaterials.2014.05.033
108. Chen ST, Wu CY, Chen HY. Enhanced growth activities of stem cell spheroids based on a durable and chemically defined surface modification coating. ACS Appl Mater Interfaces . (2018) 10:31882–91. doi: 10.1021/acsami.8b09103
109. Hu W, Zhu S, Fanai ML, Wang J, Cai J, Feng J. 3d co-culture model of endothelial colony-forming cells (Ecfcs) reverses late passage adipose-derived stem cell senescence for wound healing. Stem Cell Res Ther . (2020) 11:355. doi: 10.1186/s13287-020-01838-w
110. Lin IC, Wang TJ, Wu CL, Lu DH, Chen YR, Yang KC. Chitosan-cartilage extracellular matrix hybrid scaffold induces chondrogenic differentiation to adipose-derived stem cells. Regener Ther . (2020) 14:238–44. doi: 10.1016/j.reth.2020.03.014
111. Kim G, Jung Y, Cho K, Lee HJ, Koh WG. Thermoresponsive poly(N-isopropylacrylamide) hydrogel substrates micropatterned with poly(Ethylene glycol) hydrogel for adipose mesenchymal stem cell spheroid formation and retrieval. Mater Sci Eng C Mater Biol Appl . (2020) 115:111128. doi: 10.1016/j.msec.2020.111128
112. Seo J, Lee JS, Lee K, Kim D, Yang K, Shin S, et al. Switchable water-adhesive, superhydrophobic palladium-layered silicon nanowires potentiate the angiogenic efficacy of human stem cell spheroids. Adv Mater . (2014) 26:7043–50. doi: 10.1002/adma.201402273
113. Zhang S, Liu P, Chen L, Wang Y, Wang Z, Zhang B. The effects of spheroid formation of adipose-derived stem cells in a microgravity bioreactor on stemness properties and therapeutic potential. Biomaterials . (2015) 41:15–25. doi: 10.1016/j.biomaterials.2014.11.019
114. Kim Y, Baipaywad P, Jeong Y, Park H. Incorporation of gelatin microparticles on the formation of adipose-derived stem cell spheroids. Int J Biol Macromol . (2018) 110:472–8. doi: 10.1016/j.ijbiomac.2018.01.046
115. Wu Y, Hospodiuk M, Peng W, Gudapati H, Neuberger T, Koduru S, et al. Porous tissue strands: avascular building blocks for scalable tissue fabrication. Biofabrication . (2018) 11:015009. doi: 10.1088/1758-5090/aaec22
116. Kim SJ, Park J, Byun H, Park YW, Major LG, Lee DY, et al. Hydrogels with an embossed surface: an all-in-one platform for mass production and culture of human adipose-derived stem cell spheroids. Biomaterials . (2019) 188:198–212. doi: 10.1016/j.biomaterials.2018.10.025
117. Tsai CC, Hong YJ, Lee RJ, Cheng NC, Yu J. Enhancement of human adipose-derived stem cell spheroid differentiation in an in situ enzyme-crosslinked gelatin hydrogel. J Mater Chem B . (2019) 7:1064–75. doi: 10.1039/C8TB02835D
118. Zhao Y, Xiao E, Lv W, Dong X, He L, Wang Y, et al. A chemically defined serum-free culture system for spontaneous human mesenchymal stem cell spheroid formation. Stem Cells Int . (2020) 2020:1031985. doi: 10.1155/2020/1031985
119. Labriola NR, Sadick JS, Morgan JR, Mathiowitz E, Darling EM. Cell mimicking microparticles influence the organization, growth, and mechanophenotype of stem cell spheroids. Ann BioMed Eng . (2018) 46:1146–59. doi: 10.1007/s10439-018-2028-4
120. Dubey NK, Mishra VK, Dubey R, Deng YH, Tsai FC, Deng WP. Revisiting the advances in isolation, characterization and secretome of adipose-derived stromal/stem cells. Int J Mol Sci . (2018) 19:2200. doi: 10.3390/ijms19082200
121. Cheung HK, Han TT, Marecak DM, Watkins JF, Amsden BG, Flynn LE. Composite hydrogel scaffolds incorporating decellularized adipose tissue for soft tissue engineering with adipose-derived stem cells. Biomaterials . (2014) 35:1914–23. doi: 10.1016/j.biomaterials.2013.11.067
122. Mohiuddin OA, O'Donnell BT, Poche JN, Iftikhar R, Wise RM, Motherwell JM, et al. Human adipose-derived hydrogel characterization based on in vitro asc biocompatibility and differentiation. Stem Cells Int . (2019) 2019:9276398. doi: 10.1155/2019/9276398
123. Hoefner C, Muhr C, Horder H, Wiesner M, Wittmann K, Lukaszyk D, et al. Human adipose-derived mesenchymal stromal/stem cell spheroids possess high adipogenic capacity and acquire an adipose tissue-like extracellular matrix pattern. Tissue Eng Part A . (2020) 26:915–26. doi: 10.1089/ten.TEA.2019.0206
124. Rumiński S, Kalaszczyńska I, Długosz A, Lewandowska-Szumieł M. Osteogenic differentiation of human adipose-derived stem cells in 3d conditions - comparison of spheroids and polystyrene scaffolds. Eur Cell Mater . (2019) 37:382–401. doi: 10.22203/eCM.v037a23
125. Fitzgerald SJ, Cobb JS, Janorkar AV. Comparison of the formation, adipogenic maturation, and retention of human adipose-derived stem cell spheroids in scaffold-free culture techniques. J BioMed Mater Res B Appl Biomater . (2020) 108:3022–32. doi: 10.1002/jbm.b.34631
126. Turner PA, Gurumurthy B, Bailey JL, Elks CM, Janorkar AV. Adipogenic differentiation of human adipose-derived stem cells grown as spheroids. Process Biochem . (2017) 59:312–20. doi: 10.1016/j.procbio.2017.02.003
127. Yoon HH, Bhang SH, Shin JY, Shin J, Kim BS. Enhanced cartilage formation via three-dimensional cell engineering of human adipose-derived stem cells. Tissue Eng Part A . (2012) 18:1949–56. doi: 10.1089/ten.TEA.2011.0647
128. Ko JY, Park JW, Kim J, Im GI. Characterization of adipose-derived stromal/stem cell spheroids versus single-cell suspension in cell survival and arrest of osteoarthritis progression. J BioMed Mater Res A . (2021) 109:869–78. doi: 10.1002/jbm.a.37078
129. Gurumurthy B, Bierdeman PC, Janorkar AV. Spheroid model for functional osteogenic evaluation of human adipose derived stem cells. J BioMed Mater Res A . (2017) 105:1230–6. doi: 10.1002/jbm.a.35974
130. Kim BC, Kwack KH, Chun J, Lee JH. Comparative transcriptome analysis of human adipose-derived stem cells undergoing osteogenesis in 2d and 3d culture conditions. Int J Mol Sci . (2021) 22:7939. doi: 10.3390/ijms22157939
131. Cheng NC, Wang S, Young TH. The influence of spheroid formation of human adipose-derived stem cells on chitosan films on stemness and differentiation capabilities. Biomaterials . (2012) 33:1748–58. doi: 10.1016/j.biomaterials.2011.11.049
132. Amirpour N, Razavi S, Esfandiari E, Hashemibeni B, Kazemi M, Salehi H. Hanging drop culture enhances differentiation of human adipose-derived stem cells into anterior neuroectodermal cells using small molecules. Int J Dev Neurosci . (2017) 59:21–30. doi: 10.1016/j.ijdevneu.2017.03.002
133. Salehi H, Razavi S, Esfandiari E, Kazemi M, Amini S, Amirpour N. Application of hanging drop culture for retinal precursor-like cells differentiation of human adipose-derived stem cells using small molecules. J Mol Neurosci . (2019) 69:597–607. doi: 10.1007/s12031-019-01388-8
134. Bagheri-Hosseinabadi Z, Seyedi F, Mollaei HR, Moshrefi M, Seifalian A. Combination of 5-azaytidine and hanging drop culture convert fat cell into cardiac cell. Biotechnol Appl Biochem . (2021) 68:92–101. doi: 10.1002/bab.1897
135. Furuhata Y, Kikuchi Y, Tomita S, Yoshimoto K. Small spheroids of adipose-derived stem cells with time-dependent enhancement of il-8 and vegf-a secretion. Genes Cells . (2016) 21:1380–6. doi: 10.1111/gtc.12448
136. Fuku A, Taki Y, Nakamura Y, Kitajima H, Takaki T, Koya T, et al. Evaluation of the usefulness of human adipose-derived stem cell spheroids formed using spherering(®) and the lethal damage sensitivity to synovial fluid in vitro. Cells . (2022) 11:337. doi: 10.3390/cells11030337
137. Zhang J, Li C, Meng F, Guan Y, Zhang T, Yang B, et al. Functional tissue-engineered microtissue formed by self-aggregation of cells for peripheral nerve regeneration. Stem Cell Res Ther . (2022) 13:3. doi: 10.1186/s13287-021-02676-0
138. Zhou F, Hui Y, Xin H, Xu YD, Lei HE, Yang BC, et al. Therapeutic effects of adipose-derived stem cells-based microtissues on erectile dysfunction in streptozotocin-induced diabetic rats. Asian J Androl . (2017) 19:91–7. doi: 10.4103/1008-682x.182817
139. Yan D, Song Y, Zhang B, Cao G, Zhou H, Li H, et al. Progress and application of adipose-derived stem cells in the treatment of diabetes and its complications. Stem Cell Res Ther . (2024) 15:3. doi: 10.1186/s13287-023-03620-0
140. Vanikar AV, Dave SD, Thakkar UG, Trivedi HL. Cotransplantation of adipose tissue-derived insulin-secreting mesenchymal stem cells and hematopoietic stem cells: A novel therapy for insulin-dependent diabetes mellitus. Stem Cells Int . (2010) 2010:582382. doi: 10.4061/2010/582382
141. Dave SD, Vanikar AV, Trivedi HL, Thakkar UG, Gopal SC, Chandra T. Novel therapy for insulin-dependent diabetes mellitus: infusion of in vitro-generated insulin-secreting cells. Clin Exp Med . (2015) 15:41–5. doi: 10.1007/s10238-013-0266-1
142. Thakkar UG, Trivedi HL, Vanikar AV, Dave SD. Insulin-secreting adipose-derived mesenchymal stromal cells with bone marrow-derived hematopoietic stem cells from autologous and allogenic sources for type 1 diabetes mellitus. Cytotherapy . (2015) 17:940–7. doi: 10.1016/j.jcyt.2015.03.608
143. Araujo DB, Dantas JR, Silva KR, Souto DL, Pereira MFC, Moreira JP, et al. Allogenic adipose tissue-derived stromal/stem cells and vitamin D supplementation in patients with recent-onset type 1 diabetes mellitus: A 3-month follow-up pilot study. Front Immunol . (2020) 11:993. doi: 10.3389/fimmu.2020.00993
144. Dantas JR, Araújo DB, Silva KR, Souto DL, de Fátima Carvalho Pereira M, Luiz RR, et al. Adipose tissue-derived stromal/stem cells + Cholecalciferol: A pilot study in recent-onset type 1 diabetes patients. Arch Endocrinol Metab . (2021) 65:342–51. doi: 10.20945/2359-3997000000368
145. Nilforoushzadeh MA, Sisakht MM, Amirkhani MA, Seifalian AM, Banafshe HR, Verdi J, et al. Engineered skin graft with stromal vascular fraction cells encapsulated in fibrin-collagen hydrogel: A clinical study for diabetic wound healing. J Tissue Eng Regener Med . (2020) 14:424–40. doi: 10.1002/term.3003
146. Moon KC, Suh HS, Kim KB, Han SK, Young KW, Lee JW, et al. Potential of allogeneic adipose-derived stem cell-hydrogel complex for treating diabetic foot ulcers. Diabetes . (2019) 68:837–46. doi: 10.2337/db18-0699
147. Carstens MH, Quintana FJ, Calderwood ST, Sevilla JP, Ríos AB, Rivera CM, et al. Treatment of Chronic Diabetic Foot Ulcers with Adipose-Derived Stromal Vascular Fraction Cell Injections: Safety and Evidence of Efficacy at 1 year. Stem Cells Transl Med . (2021) 10:1138–47. doi: 10.1002/sctm.20-0497
148. Soria-Juan B, Garcia-Arranz M, Llanos Jiménez L, Aparicio C, Gonzalez A, Mahillo Fernandez I, et al. Efficacy and safety of intramuscular administration of allogeneic adipose tissue derived and expanded mesenchymal stromal cells in diabetic patients with critical limb ischemia with no possibility of revascularization: study protocol for a randomized controlled double-blind phase ii clinical trial (the noma trial). Trials . (2021) 22:595. doi: 10.1186/s13063-021-05430-2
149. Nuschke A. Activity of mesenchymal stem cells in therapies for chronic skin wound healing. Organogenesis . (2014) 10:29–37. doi: 10.4161/org.27405
150. Ianus A, Holz GG, Theise ND, Hussain MA. In vivo derivation of glucose-competent pancreatic endocrine cells from bone marrow without evidence of cell fusion. J Clin Invest . (2003) 111:843–50. doi: 10.1172/jci16502
151. American Diabetes Association. Diagnosis and classification of diabetes mellitus. Diabetes Care . (2009) 32(Suppl 1):S62–7. doi: 10.2337/dc09-S062
152. Atkinson MA, Eisenbarth GS, Michels AW. Type 1 diabetes. Lancet . (2014) 383:69–82. doi: 10.1016/s0140-6736(13)60591-7
153. Mohandas S, Gayatri V, Kumaran K, Gopinath V, Paulmurugan R, Ramkumar KM. New frontiers in three-dimensional culture platforms to improve diabetes research. Pharmaceutics . (2023) 15:725. doi: 10.3390/pharmaceutics15030725
154. Khorsandi L, Khodadadi A, Nejad-Dehbashi F, Saremy S. Three-dimensional differentiation of adipose-derived mesenchymal stem cells into insulin-producing cells. Cell Tissue Res . (2015) 361:745–53. doi: 10.1007/s00441-015-2140-9
155. Ikemoto T, Feng R, Iwahashi SI, Yamada S, Saito Y, Morine Y, et al. In vitro and in vivo effects of insulin-producing cells generated by xeno-antigen free 3d culture with rcp piece. Sci Rep . (2019) 9:10759. doi: 10.1038/s41598-019-47257-7
156. Ohta S, Ikemoto T, Wada Y, Saito Y, Yamada S, Imura S, et al. A change in the zinc ion concentration reflects the maturation of insulin-producing cells generated from adipose-derived mesenchymal stem cells. Sci Rep . (2019) 9:18731. doi: 10.1038/s41598-019-55172-0
157. Abadpour S, Niemi EM, Orrhult LS, Hermanns C, de Vries R, Nogueira LP, et al. Adipose-derived stromal cells preserve pancreatic islet function in a transplantable 3d bioprinted scaffold. Adv Healthc Mater . (2023) 12:e2300640. doi: 10.1002/adhm.202300640
158. Wang X, Jin L, Liu W, Stingelin L, Zhang P, Tan Z. Construction of engineered 3d islet micro-tissue using porcine decellularized ecm for the treatment of diabetes. Biomater Sci . (2023) 11:5517–32. doi: 10.1039/D3BM00346A
159. Zhao X, Guo J, Zhang F, Zhang J, Liu D, Hu W, et al. Therapeutic application of adipose-derived stromal vascular fraction in diabetic foot. Stem Cell Res Ther . (2020) 11:394. doi: 10.1186/s13287-020-01825-1
160. Gurtner GC, Werner S, Barrandon Y, Longaker MT. Wound repair and regeneration. Nature . (2008) 453:314–21. doi: 10.1038/nature07039
161. Wicks K, Torbica T, Mace KA. Myeloid cell dysfunction and the pathogenesis of the diabetic chronic wound. Semin Immunol . (2014) 26:341–53. doi: 10.1016/j.smim.2014.04.006
162. Armstrong DG, Tan TW, Boulton AJM, Bus SA. Diabetic foot ulcers: A review. Jama . (2023) 330:62–75. doi: 10.1001/jama.2023.10578
163. Davis FM, Kimball A, Boniakowski A, Gallagher K. Dysfunctional wound healing in diabetic foot ulcers: New crossroads. Curr Diabetes Rep . (2018) 18:2. doi: 10.1007/s11892-018-0970-z
164. Lipsky BA. Diabetic foot infections: Current treatment and delaying the 'Post-antibiotic era'. Diabetes Metab Res Rev . (2016) 32 Suppl 1:246–53. doi: 10.1002/dmrr.2739
165. Liu R, Dong R, Chang M, Liang X, Wang HC. Adipose-derived stem cells for the treatment of diabetic wound: From basic study to clinical application. Front Endocrinol (Lausanne) . (2022) 13:882469. doi: 10.3389/fendo.2022.882469
166. Schneider I, Calcagni M, Buschmann J. Adipose-derived stem cells applied in skin diseases, wound healing and skin defects: A review. Cytotherapy . (2023) 25:105–19. doi: 10.1016/j.jcyt.2022.08.005
167. Lee DE, Ayoub N, Agrawal DK. Mesenchymal stem cells and cutaneous wound healing: Novel methods to increase cell delivery and therapeutic efficacy. Stem Cell Res Ther . (2016) 7:37. doi: 10.1186/s13287-016-0303-6
168. Turner NJ, Badylak SF. The use of biologic scaffolds in the treatment of chronic nonhealing wounds. Adv Wound Care (New Rochelle) . (2015) 4:490–500. doi: 10.1089/wound.2014.0604
169. Zeng Y, Zhu L, Han Q, Liu W, Mao X, Li Y, et al. Preformed gelatin microcryogels as injectable cell carriers for enhanced skin wound healing. Acta Biomater . (2015) 25:291–303. doi: 10.1016/j.actbio.2015.07.042
170. Feng J, Mineda K, Wu SH, Mashiko T, Doi K, Kuno S, et al. An injectable non-cross-linked hyaluronic-acid gel containing therapeutic spheroids of human adipose-derived stem cells. Sci Rep . (2017) 7:1548. doi: 10.1038/s41598-017-01528-3
171. Tyeb S, Shiekh PA, Verma V, Kumar A. Adipose-derived stem cells (Adscs) loaded gelatin-sericin-laminin cryogels for tissue regeneration in diabetic wounds. Biomacromolecules . (2020) 21:294–304. doi: 10.1021/acs.biomac.9b01355
172. Amos PJ, Kapur SK, Stapor PC, Shang H, Bekiranov S, Khurgel M, et al. Human adipose-derived stromal cells accelerate diabetic wound healing: Impact of cell formulation and delivery. Tissue Eng Part A . (2010) 16:1595–606. doi: 10.1089/ten.TEA.2009.0616
173. Lee JS, Chae S, Yoon D, Yoon D, Chun W, Kim GH. Angiogenic factors secreted from human asc spheroids entrapped in an alginate-based hierarchical structure via combined 3d printing/electrospinning system. Biofabrication . (2020) 12:045028. doi: 10.1088/1758-5090/abaf9a
174. Gerlach JC, Lin YC, Brayfield CA, Minteer DM, Li H, Rubin JP, et al. Adipogenesis of human adipose-derived stem cells within three-dimensional hollow fiber-based bioreactors. Tissue Eng Part C Methods . (2012) 18:54–61. doi: 10.1089/ten.TEC.2011.0216
175. Yang F, Carmona A, Stojkova K, Garcia Huitron EI, Goddi A, Bhushan A, et al. A 3d human adipose tissue model within a microfluidic device. Lab Chip . (2021) 21:435–46. doi: 10.1039/D0LC00981D
176. Tharp KM, Stahl A. Bioengineering beige adipose tissue therapeutics. Front Endocrinol (Lausanne) . (2015) 6:164. doi: 10.3389/fendo.2015.00164
177. Tharp KM, Jha AK, Kraiczy J, Yesian A, Karateev G, Sinisi R, et al. Matrix-assisted transplantation of functional beige adipose tissue. Diabetes . (2015) 64:3713–24. doi: 10.2337/db15-0728
178. Yang JP, Anderson AE, McCartney A, Ory X, Ma G, Pappalardo E, et al. Metabolically active three-dimensional brown adipose tissue engineered from white adipose-derived stem cells. Tissue Eng Part A . (2017) 23:253–62. doi: 10.1089/ten.TEA.2016.0399
179. Lau FH, Vogel K, Luckett JP, Hunt M, Meyer A, Rogers CL, et al. Sandwiched white adipose tissue: A microphysiological system of primary human adipose tissue. Tissue Eng Part C Methods . (2018) 24:135–45. doi: 10.1089/ten.TEC.2017.0339
180. Kang JH, Gimble JM, Kaplan DL. In vitro 3d model for human vascularized adipose tissue. Tissue Eng Part A . (2009) 15:2227–36. doi: 10.1089/ten.tea.2008.0469
181. Paek J, Park SE, Lu Q, Park KT, Cho M, Oh JM, et al. Microphysiological engineering of self-assembled and perfusable microvascular beds for the production of vascularized three-dimensional human microtissues. ACS Nano . (2019) 13:7627–43. doi: 10.1021/acsnano.9b00686
182. Kim SJ, Byun H, Lee S, Kim E, Lee GM, Huh SJ, et al. Spatially arranged encapsulation of stem cell spheroids within hydrogels for the regulation of spheroid fusion and cell migration. Acta Biomater . (2022) 142:60–72. doi: 10.1016/j.actbio.2022.01.047
183. Shen K, Vesey DA, Hasnain SZ, Zhao KN, Wang H, Johnson DW, et al. A cost-effective three-dimensional culture platform functionally mimics the adipose tissue microenvironment surrounding the kidney. Biochem Biophys Res Commun . (2020) 522:736–42. doi: 10.1016/j.bbrc.2019.11.119
184. Yu J, Hsu YC, Lee JK, Cheng NC. Enhanced angiogenic potential of adipose-derived stem cell sheets by integration with cell spheroids of the same source. Stem Cell Res Ther . (2022) 13:276. doi: 10.1186/s13287-022-02948-3
185. Leong J, Hong YT, Wu YF, Ko E, Dvoretskiy S, Teo JY, et al. Surface tethering of inflammation-modulatory nanostimulators to stem cells for ischemic muscle repair. ACS Nano . (2020) 14:5298–313. doi: 10.1021/acsnano.9b04926
186. Jiao W, Liu C, Shan J, Kong Z, Wang X. Construction and evaluation of small-diameter bioartificial arteries based on a combined-mold technology. Polymers (Basel) . (2022) 14:3089. doi: 10.3390/polym14153089
187. Fu H, Zhang D, Zeng J, Fu Q, Chen Z, Sun X, et al. Application of 3d-printed tissue-engineered skin substitute using innovative biomaterial loaded with human adipose-derived stem cells in wound healing. Int J Bioprint . (2023) 9:674. doi: 10.18063/ijb.v9i2.674
188. Li M, Ma J, Gao Y, Dong M, Zheng Z, Li Y, et al. Epithelial differentiation of human adipose-derived stem cells (Hascs) undergoing three-dimensional (3d) cultivation with collagen sponge scaffold (Css) via an indirect co-culture strategy. Stem Cell Res Ther . (2020) 11:141. doi: 10.1186/s13287-020-01645-3
189. Alamán-Díez P, García-Gareta E, Arruebo M, Pérez M. A bone-on-a-chip collagen hydrogel-based model using pre-differentiated adipose-derived stem cells for personalized bone tissue engineering. J BioMed Mater Res A . (2023) 111:88–105. doi: 10.1002/jbm.a.37448
190. Sun W, Zhang J, Qin Y, Tang H, Chen Y, Lin W, et al. A simple and efficient strategy for preparing a cell-spheroid-based bioink. Adv Healthc Mater . (2022) 11:e2200648. doi: 10.1002/adhm.202200648
191. Lee J, Lee S, Huh SJ, Kang BJ, Shin H. Directed regeneration of osteochondral tissue by hierarchical assembly of spatially organized composite spheroids. Adv Sci (Weinh) . (2022) 9:e2103525. doi: 10.1002/advs.202103525
192. Fujimoto R, Murata D, Nakayama K. Bio-3d printing of scaffold-free osteogenic and chondrogenic constructs using rat adipose-derived stromal cells. Front Biosci (Landmark Ed) . (2022) 27:52. doi: 10.31083/j.fbl2702052
193. Celik N, Kim MH, Yeo M, Kamal F, Hayes DJ, Ozbolat IT. Mirna induced 3d bioprinted-heterotypic osteochondral interface. Biofabrication . (2022) 14:10. doi: 10.1088/1758-5090/ac7fbb
194. Landau S, Szklanny AA, Machour M, Kaplan B, Shandalov Y, Redenski I, et al. Human-engineered auricular reconstruction (Hear) by 3d-printed molding with human-derived auricular and costal chondrocytes and adipose-derived mesenchymal stem cells. Biofabrication . (2021) 14:1–14. doi: 10.1088/1758-5090/ac3b91
195. Jang CH, Koo Y, Kim G. Asc/chondrocyte-laden alginate hydrogel/pcl hybrid scaffold fabricated using 3d printing for auricle regeneration. Carbohydr Polym . (2020) 248:116776. doi: 10.1016/j.carbpol.2020.116776
196. Ibrahim A, Rodriguez-Florez N, Gardner OFW, Zucchelli E, New SEP, Borghi A, et al. Three-dimensional environment and vascularization induce osteogenic maturation of human adipose-derived stem cells comparable to that of bone-derived progenitors. Stem Cells Transl Med . (2020) 9:1651–66. doi: 10.1002/sctm.19-0207
197. Ewa-Choy YW, Pingguan-Murphy B, Abdul-Ghani NA, Jahendran J, Chua KH. Effect of alginate concentration on chondrogenesis of co-cultured human adipose-derived stem cells and nasal chondrocytes: A biological study. Biomater Res . (2017) 21:19. doi: 10.1186/s40824-017-0105-7
198. Guerrero J, Pigeot S, Müller J, Schaefer DJ, Martin I, Scherberich A. Fractionated human adipose tissue as a native biomaterial for the generation of a bone organ by endochondral ossification. Acta Biomater . (2018) 77:142–54. doi: 10.1016/j.actbio.2018.07.004
199. Ergene E, Sezlev Bilecen D, Kaya B, Yilgor Huri P, Hasirci V. 3d cellular alignment and biomimetic mechanical stimulation enhance human adipose-derived stem cell myogenesis. BioMed Mater . (2020) 15:055017. doi: 10.1088/1748-605X/ab95e2
200. Liu BH, Yeh HY, Lin YC, Wang MH, Chen DC, Lee BH, et al. Spheroid formation and enhanced cardiomyogenic potential of adipose-derived stem cells grown on chitosan. Biores Open Access . (2013) 2:28–39. doi: 10.1089/biores.2012.0285
201. Gomila Pelegri N, Stanczak AM, Bottomley AL, Cummins ML, Milthorpe BK, Gorrie CA, et al. Neural marker expression in adipose-derived stem cells grown in peg-based 3d matrix is enhanced in the presence of B27 and cultureone supplements. Int J Mol Sci . (2023) 24:16269. doi: 10.3390/ijms242216269
202. Wang Y, Lee JH, Shirahama H, Seo J, Glenn JS, Cho NJ. Extracellular matrix functionalization and huh-7.5 cell coculture promote the hepatic differentiation of human adipose-derived mesenchymal stem cells in a 3d icc hydrogel scaffold. ACS Biomater Sci Eng . (2016) 2:2255–65. doi: 10.1021/acsbiomaterials.6b00487
203. Scioli MG, Storti G, D'Amico F, Gentile P, Kim BS, Cervelli V, et al. Adipose-derived stem cells in cancer progression: New perspectives and opportunities. Int J Mol Sci . (2019) 20:3296. doi: 10.3390/ijms20133296
204. Strong AL, Burow ME, Gimble JM, Bunnell BA. Concise review: The obesity cancer paradigm: exploration of the interactions and crosstalk with adipose stem cells. Stem Cells . (2015) 33:318–26. doi: 10.1002/stem.1857
205. Freese KE, Kokai L, Edwards RP, Philips BJ, Sheikh MA, Kelley J, et al. Adipose-derived stems cells and their role in human cancer development, growth, progression, and metastasis: A systematic review. Cancer Res . (2015) 75:1161–8. doi: 10.1158/0008-5472.Can-14-2744
206. Ko SF, Yip HK, Zhen YY, Lee CC, Lee CC, Huang CC, et al. Adipose-derived mesenchymal stem cell exosomes suppress hepatocellular carcinoma growth in a rat model: apparent diffusion coefficient, natural killer T-cell responses, and histopathological features. Stem Cells Int . (2015) 2015:853506. doi: 10.1155/2015/853506
207. Chuang CC, Chen YN, Wang YY, Huang YC, Lin SY, Huang RY, et al. Stem cell-based delivery of gold/chlorin E6 nanocomplexes for combined photothermal and photodynamic therapy. ACS Appl Mater Interfaces . (2020) 12:30021–30. doi: 10.1021/acsami.0c03446
Keywords: adipose-derived stem/stromal cells, monolayer culture, three-dimensional culture, diabetes mellitus, diabetic foot ulcer
Citation: Shi Y, Yang X, Min J, Kong W, Hu X, Zhang J and Chen L (2024) Advancements in culture technology of adipose-derived stromal/stem cells: implications for diabetes and its complications. Front. Endocrinol. 15:1343255. doi: 10.3389/fendo.2024.1343255
Received: 23 November 2023; Accepted: 29 March 2024; Published: 12 April 2024.
Reviewed by:
Copyright © 2024 Shi, Yang, Min, Kong, Hu, Zhang and Chen. This is an open-access article distributed under the terms of the Creative Commons Attribution License (CC BY) . The use, distribution or reproduction in other forums is permitted, provided the original author(s) and the copyright owner(s) are credited and that the original publication in this journal is cited, in accordance with accepted academic practice. No use, distribution or reproduction is permitted which does not comply with these terms.
*Correspondence: Lulu Chen, [email protected] ; Jiaoyue Zhang, [email protected]
† These authors have contributed equally to this work
Disclaimer: All claims expressed in this article are solely those of the authors and do not necessarily represent those of their affiliated organizations, or those of the publisher, the editors and the reviewers. Any product that may be evaluated in this article or claim that may be made by its manufacturer is not guaranteed or endorsed by the publisher.
Enhancing insulin sensitivity in type 2 diabetes mellitus using apelin-loaded small extracellular vesicles from Wharton's jelly-derived mesenchymal stem cells: a novel therapeutic approach
Affiliations.
- 1 The Fifth School of Clinical Medicine, Navy Clinical College, Anhui Medical University, Hefei, Anhui, China.
- 2 Department of Cardiology, The Sixth Medical Center of Chinese People's Liberation Army General Hospital, Beijing, China·, China.
- 3 Department of Dermatology, Peking University Third Hospital, Beijing, China.
- 4 Department of Cardiology, The Sixth Medical Center of Chinese People's Liberation Army General Hospital, Beijing, China·, China. [email protected].
- 5 The Fifth School of Clinical Medicine, Navy Clinical College, Anhui Medical University, Hefei, Anhui, China. [email protected].
- 6 Department of Cardiology, The Sixth Medical Center of Chinese People's Liberation Army General Hospital, Beijing, China·, China. [email protected].
- PMID: 38622732
- PMCID: PMC11020616
- DOI: 10.1186/s13098-024-01332-w
Background: Type 2 diabetes mellitus (T2DM), characterized by β-cell dysfunction and insulin resistance (IR), presents considerable treatment challenges. Apelin is an adipocyte-derived factor that shows promise in improving IR; however, it is limited by poor targeting and a short half-life. In the present study, engineered small extracellular vesicles (sEVs) derived from Wharton's jelly-derived mesenchymal stem cells (WJ-MSCs) loaded with apelin were used to address the limitations of the therapeutic application of apelin.
Methods: WJ-MSCs were transduced to obtain engineered sEVs loaded with overexpressed apelin (apelin-MSC-sEVs) and the control sEVs (MSC-sEVs). T2DM mice were injected with apelin-MSC-sEVs and MSC-sEVs, and blood glucose monitoring, glucose and insulin tolerance tests, confocal microscopy, and immunocytochemical analysis were performed. IR models of 3T3-L1 adipocytes were employed to detect GLUT4 expression in each group using western blotting; the affected pathways were determined by measuring the changes in Akt and AMPK signaling and phosphorylation.
Results: Upon successful engineering, WJ-MSCs demonstrated significant overexpression of apelin. The genetic modification did not adversely impact the characteristics of sEVs, ranging from surface protein markers, morphology, to particle size, but generated apelin-overexpressed sEVs. Apelin-MSC-sEVs treatment resulted in notable enhancement of Akt and AMPK pathway activities within 3T3-L1 adipocytes and adipose tissues of T2DM mice. Furthermore, the apelin-loaded sEVs significantly reduced plasma glucose levels, increased pancreatic β-cell proliferation, improved insulin and glucose tolerance, and modulated pro-inflammatory cytokine profiles, compared to mice treated with the control sEVs.
Conclusion: Our study developed novel genetically engineered apelin-loaded sEVs derived from WJ-MSCs, and demonstrated their potent role in augmenting insulin sensitivity and regulating inflammatory responses, highlighting their therapeutic promise in T2DM management. The findings open new avenues for the development of clinically viable treatments for T2DM in humans using the apelin-loaded sEVs.
Keywords: Apelin; IR; Small extracellular vesicles; Type 2 diabetes mellitus; Wharton’s jelly-derived mesenchymal stem cells.
© 2024. The Author(s).
Grants and funding
- 2022YFA1104300/National Key Research and Development Program of China
- 7222183/Beijing Municipal Natural Science Foundation
- 2022YFA1104300/Anhui Province Graduate Student Quality Engineering Program
- U.S. Department of Health & Human Services

- Virtual Tour
- Staff Directory
- En Español
You are here
Nih research matters.
April 16, 2024
Rejuvenating the immune system by depleting certain stem cells
At a glance.
- Researchers found that depleting certain stem cells improved the immune systems of aged mice.
- The findings suggest that a similar treatment might be used to help protect older people against infections.

The risk for serious infections rises with age, as people’s immune systems lose the ability to respond to novel infections. Part of the reason for this is that the types of hematopoietic stem cells (HSCs), which make the various types of blood cells, change with age.
Some HSCs, called myeloid-biased HSCs (my-HSCs) produce mostly myeloid cells, which include red blood cells, platelets, and most cells of the innate immune system. Others, called balanced HSCs (bal-HSCs), produce a healthy mix of myeloid and lymphoid cells, which include the T and B cells that make up the adaptive immune system.
The proportion of my-HSCs increases with age. This leads to more myeloid cells and fewer lymphoid cells. More myeloid cells increase inflammation and bring an increased risk of atherosclerosis and myeloid-related diseases such as leukemia. Fewer lymphoid cells reduces the ability to fight infections. A research team led by Drs. Kim Hasenkrug and Lara Myers at NIH and Drs. Irving Weissman and Jason Ross at Stanford University School of Medicine explored whether reducing my-HSCs could restore a more “youthful” immune system in aged mice. The results appeared in Nature on March 27, 2024.
The team began by identifying proteins on the surface of mouse HSCs that are unique to my-HSCs. They then created antibodies against these proteins and used them to deplete my-HSCs in aged mice.
Depleting my-HSCs reduced the effects of aging on the mouse immune system. It increased lymphoid progenitor cells, which give rise to T and B cells, in the bone marrow. Consequently, treated mice had more naïve T cells and B cells in their blood than untreated mice. These cells allow the immune system to learn to recognize novel infections. The treatment also lowered levels of exhausted T cells and age-associated B cells, along with certain inflammatory markers.
When the researchers vaccinated aged mice with a live, weakened virus, those with depleted my-HSCs had a stronger T cell response than untreated mice. The treated mice also gained better protection against infection from the vaccination.
These findings could explain why older people are more vulnerable to infections such as SARS-CoV-2. Weakened adaptive immunity from fewer lymphoid cells makes it harder for them to fight off the infection. At the same time, increased myeloid cells cause harmful inflammation. The researchers noted that the genes that characterize my-HSCs in mice are also found in aged human HSCs. This suggests that my-HSC depletion might be used in humans to relieve certain age-associated health problems.
“During the start of the COVID-19 pandemic, it quickly became clear that older people were dying in larger numbers than younger people,” Weissman says. “This trend continued even after vaccinations became available. If we can revitalize the aging human immune system like we did in mice, it could be lifesaving when the next global pathogen arises.”
—by Brian Doctrow, Ph.D.
Related Links
- Human Skeletal Stem Cell Identified
- Gene Therapy Reduces Need for Transfusions in Severe Blood Disorder
- Stem Cell Transplant Induces Multiple Sclerosis Remission
- Immune System Reset May Halt Multiple Sclerosis Progression
- Gene Editing Corrects Sickle Cell Mutation
- Senescent Cells Tied to Health and Longevity In Mice
- Fruit Flies Yield Insights into Aging
- Stem Cell Transplant Reverses Sickle Cell Disease in Adults
- Introduction to Stem Cells
References: Depleting myeloid-biased haematopoietic stem cells rejuvenates aged immunity. Ross JB, Myers LM, Noh JJ, Collins MM, Carmody AB, Messer RJ, Dhuey E, Hasenkrug KJ, Weissman IL. Nature . 2024 Apr;628(8006):162-170. doi: 10.1038/s41586-024-07238-x. Epub 2024 Mar 27. PMID: 38538791.
Funding: NIH’s National Institute of Allergy and Infectious Diseases (NIAID), National Cancer Institute (NCI), National Institute of Diabetes and Digestive and Kidney Diseases (NIDDK), and National Institute of General Medical Sciences (NIGMS); Virginia and D.K. Ludwig Fund for Cancer Research; Stanford University; Radiological Society of North America; Stanford Cancer Institute.
Connect with Us
- More Social Media from NIH
- Program Finder
- Admissions Services
- Course Directory
- Academic Calendar
- Hybrid Campus
- Lecture Series
- Convocation
- Strategy and Development
- Implementation and Impact
- Integrity and Oversight
- In the School
- In the Field
- In Baltimore
- Resources for Practitioners
- Articles & News Releases
- In The News
- Statements & Announcements
- At a Glance
- Student Life
- Strategic Priorities
- Inclusion, Diversity, Anti-Racism, and Equity (IDARE)
- What is Public Health?
Research Gaps Around Type 1 Diabetes
A large body of research on Type 2 diabetes has helped to develop guidance, informing how patients are diagnosed, treated, and manage their lifestyle. In contrast, Type 1 diabetes, often mistakenly associated only with childhood, has received less attention.
In this Q&A, adapted from the April 17 episode of Public Health On Call , Stephanie Desmon speaks to Johns Hopkins epidemiologists Elizabeth Selvin , PhD '04, MPH, and Michael Fang , PhD, professor and assistant professor, respectively, in the Department of Epidemiology, about recent findings that challenge common beliefs about type 1 diabetes. Their conversation touches on the misconception that it’s solely a childhood condition, the rise of adult-onset cases linked to obesity, and the necessity for tailored approaches to diagnosis and care. They also discuss insulin prices and why further research is needed on medications like Ozempic in treating Type 1 diabetes.
I want to hear about some of your research that challenges what we have long understood about Type 1 diabetes, which is no longer called childhood diabetes.
MF: Type 1 diabetes was called juvenile diabetes for the longest time, and it was thought to be a disease that had a childhood onset. When diabetes occurred in adulthood it would be type 2 diabetes. But it turns out that approximately half of the cases of Type 1 diabetes may occur during adulthood right past the age of 20 or past the age of 30.
The limitations of these initial studies are that they've been in small clinics or one health system. So, it's unclear whether it's just that particular clinic or whether it applies to the general population more broadly.
We were fortunate because the CDC has collected new data that explores Type 1 diabetes in the U.S. Some of the questions they included in their national data were, “Do you have diabetes? If you do, do you have Type 1 or Type 2? And, at what age were you diagnosed?”
With these pieces of information, we were able to characterize how the age of diagnosis of Type 1 diabetes differs in the entire U.S. population.
Are Type 1 and Type 2 diabetes different diseases?
ES: They are very different diseases and have a very different burden. My whole career I have been a Type 2 diabetes epidemiologist, and I’ve been very excited to expand work with Type 1 diabetes.
There are about 1.5 million adults with Type 1 diabetes in the U.S., compared to 21 million adults with Type 2 diabetes. In terms of the total cases of diabetes, only 5 to 10 percent have Type 1 diabetes. Even in our largest epidemiologic cohorts, only a small percentage of people have Type 1 diabetes. So, we just don't have the same national data, the same epidemiologic evidence for Type 1 diabetes that we have for Type 2. The focus of our research has been trying to understand and characterize the general epidemiology and the population burden of Type 1 diabetes.
What is it about Type 1 that makes it so hard to diagnose?
MF: The presentation of symptoms varies by age of diagnosis. When it occurs in children, it tends to have a very acute presentation and the diagnosis is easier to make. When it happens in adulthood, the symptoms are often milder and it’s often misconstrued as Type 2 diabetes.
Some studies have suggested that when Type 1 diabetes occurs in adulthood, about 40% of those cases are misdiagnosed initially as Type 2 cases. Understanding how often people get diagnosed later in life is important to correctly diagnose and treat patients.
Can you talk about the different treatments?
MF: Patients with Type 1 diabetes are going to require insulin. Type 2 diabetes patients can require insulin, but that often occurs later in the disease, as oral medications become less and less effective.
ES: Because of the epidemic of overweight and obese in the general population, we’re seeing a lot of people with Type 1 diabetes who are overweight and have obesity. This can contribute to issues around misdiagnosis because people with Type 1 diabetes will have signs and will present similarly to Type 2 diabetes. They'll have insulin resistance potentially as a result of weight gain metabolic syndrome. Some people call it double diabetes—I don't like that term—but it’s this idea that if you have Type 1 diabetes, you can also have characteristics of Type 2 diabetes as well.
I understand that Type 1 used to be considered a thin person's disease, but that’s not the case anymore. MF: In a separate paper, we also explored the issue of overweight and obesity in persons with Type 1 diabetes. We found that approximately 62% of adults with Type 1 diabetes were either overweight or obese, which is comparable to the general U.S. population.
But an important disclaimer is that weight management in this population [with Type 1 diabetes] is very different. They can't just decide to go on a diet, start jogging, or engage in rigorous exercise. It can be a very, very dangerous thing to do.
Everybody's talking about Ozempic and Mounjaro—the GLP-1 drugs—for diabetes or people who are overweight to lose weight and to solve their diabetes. Where does that fit in with this population?
ES: These medications are used to treat Type 2 diabetes in the setting of obesity. Ozempic and Mounjaro are incretin hormones. They mediate satiation, reduce appetite, slow gastric emptying, and lower energy intake. They're really powerful drugs that may be helpful in Type 1 diabetes, but they're not approved for the management of obesity and Type 1 diabetes. At the moment, there aren't data to help guide their use in people with Type 1 diabetes, but I suspect they're going to be increasingly used in people with Type 1 diabetes.
MF: The other piece of managing weight—and it's thought to be foundational for Type 1 or Type 2—is dieting and exercising. However, there isn’t good guidance on how to do this in persons with Type 1 diabetes, whereas there are large and rigorous trials in Type 2 patients. We’re really just starting to figure out how to safely and effectively manage weight with lifestyle changes for Type 1 diabetics, and I think that's an important area of research that should continue moving forward.
ES: Weight management in Type 1 diabetes is complicated by insulin use and the risk of hypoglycemia, or your glucose going too low, which can be an acute complication of exercise. In people with Type 2 diabetes, we have a strong evidence base for what works. We know modest weight loss can help prevent the progression and development of Type 2 diabetes, as well as weight gain. In Type 1, we just don't have that evidence base.
Is there a concern about misdiagnosis and mistreatment? Is it possible to think a patient has Type 2 but they actually have Type 1?
MF: I think so. Insulin is the overriding concern. In the obesity paper, we looked at the percentage of people who said their doctors recommended engaging in more exercise and dieting. We found that people with Type 1 diabetes were less likely to receive the same guidance from their doctor. I think providers may be hesitant to say, “Look, just go engage in an active lifestyle.”
This is why it's important to have those studies and have that guidance so that patients and providers can be comfortable in improving lifestyle management.
Where is this research going next?
ES: What's clear from these studies is that the burden of overweight and obesity is substantial in people with Type 1 diabetes and it's not adequately managed. Going forward, I think we're going to need clinical trials, clear clinical guidelines, and patient education that addresses how best to tackle obesity in the setting of Type 1 diabetes.
It must be confusing for people with Type 1 diabetes who are hearing about people losing all this weight on these drugs, but they go to their doctor who says, “Yeah, but that's not for you.”
ES: I hope it's being handled more sensitively. These drugs are being used by all sorts of people for whom they are not indicated, and I'm sure that people with Type 1 diabetes are accessing these drugs. I think the question is, are there real safety issues? We need thoughtful discussion about this and some real evidence to make sure that we're doing more good than harm.
MF: Dr. Selvin’s group has published a paper, estimating that about 15% of people with Type 1 diabetes are on a GLP-1. But we don't have great data on what potentially can happen to individuals.
The other big part of diabetes that we hear a lot about is insulin and its price. Can you talk about your research on this topic?
MF: There was a survey that asked, “Has there been a point during the year when you were not using insulin because you couldn’t afford it?” About 20% of adults under the age of 65 said that at some point during the year, they couldn't afford their insulin and that they did engage in what sometimes is called “cost-saving rationing” [of insulin].
Medicare is now covering cheaper insulin for those over 65, but there are a lot of people for whom affordability is an issue. Can you talk more about that?
MF: The fight is not over. Just because there are national and state policies, and now manufacturers have been implementing price caps, doesn't necessarily mean that the people who need insulin the most are now able to afford it.
A recent study in the Annals of Internal Medicine looked at states that adopted or implemented out-of-pocket cost caps for insulin versus those that didn't and how that affected insulin use over time. They found that people were paying less for insulin, but the use of insulin didn't change over time. The $35 cap is an improvement, but we need to do more.
ES: There are still a lot of formulations of insulin that are very expensive. $35 a month is not cheap for someone who is on insulin for the rest of their lives.
- Overweight and Obesity in People With Type 1 Diabetes Nearly Same as General Population
- The Impacts of COVID-19 on Diabetes and Insulin
- Why Eli Lilly’s Insulin Price Cap Announcement Matters
Related Content
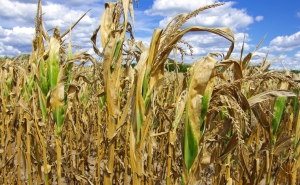
To Protect Human Health, We Must Protect the Earth’s Health
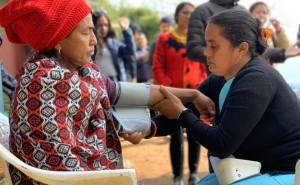
New Grant Enables Johns Hopkins Researchers to Implement Community Health Worker-Led Health Interventions for Noncommunicable Diseases in Nepal
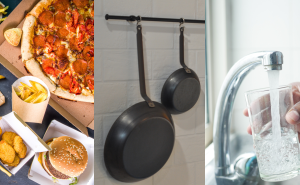
The Omnipresence of PFAS—and What We Can Do About Them
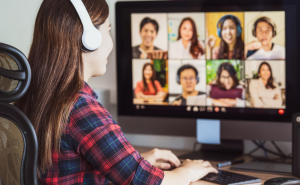
Summer Institute in Data to Policy
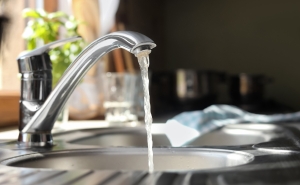
Filters and Digital Health Program Reduced Participants' Arsenic Levels by Nearly Half in American Indian Households Relying on Well Water
Thank you for visiting nature.com. You are using a browser version with limited support for CSS. To obtain the best experience, we recommend you use a more up to date browser (or turn off compatibility mode in Internet Explorer). In the meantime, to ensure continued support, we are displaying the site without styles and JavaScript.
- View all journals
- Explore content
- About the journal
- Publish with us
- Sign up for alerts
- 14 July 2021
How stem cells could fix type 1 diabetes
- Liam Drew 0
Liam Drew is a freelance writer near London.
You can also search for this author in PubMed Google Scholar
Insulin has been one of the most transformative discoveries in medicine. The isolation of this hormone in 1921 made type 1 diabetes (T1D) a treatable, rather than a terminal, illness. However, there is growing hope that 100 years later, insulin therapy for T1D might be on the brink of obsolescence.
Access options
Access Nature and 54 other Nature Portfolio journals
Get Nature+, our best-value online-access subscription
24,99 € / 30 days
cancel any time
Subscribe to this journal
Receive 51 print issues and online access
185,98 € per year
only 3,65 € per issue
Rent or buy this article
Prices vary by article type
Prices may be subject to local taxes which are calculated during checkout
Nature 595 , S64-S66 (2021)
doi: https://doi.org/10.1038/d41586-021-01842-x
This article is part of Nature Outlook: Autoimmune disease , an editorially independent supplement produced with the financial support of third parties. About this content .
Katsarou, A. et al. Nature Rev. Dis. Primers 3 , 17016 (2017).
Article PubMed Google Scholar
Gamble, A., Pepper, A. R., Bruni, A. & Shapiro, A. M. J. Islets 10 , 80–94 (2018).
Shapiro, A. M. J. et al. N. Engl. J. Med. 343 , 230–238 (2000).
Pagliuca, F. W. et al. Cell 159 , 428–439 (2014).
Rezania, A. et al. Nature Biotechnol. 32 , 1121–1133 (2014).
D’Amour, K. A. et al. Nature Biotechnol. 24 , 1392–1401 (2006).
Kroon, E. et al. Nature Biotechnol. 26 , 443–452 (2008).
Stock, A. A. et al. Stem Cell Rep. 14 , 91–104 (2020).
Article Google Scholar
Download references
Related Articles
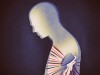
- Regeneration
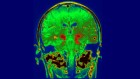
Diabetes drug slows development of Parkinson’s disease
News 03 APR 24
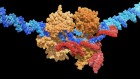
Stealthy stem cells to treat disease
Spotlight 28 FEB 24
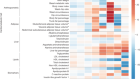
Genetic drivers of heterogeneity in type 2 diabetes pathophysiology
Article 19 FEB 24
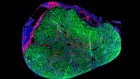
‘Mini liver’ will grow in person’s own lymph node in bold new trial
News 02 APR 24
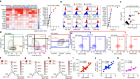
Depleting myeloid-biased haematopoietic stem cells rejuvenates aged immunity
Article 27 MAR 24
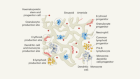
Powerful microscopy reveals blood-cell production in bone marrow
News & Views 20 MAR 24
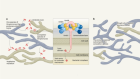
Bacteria deploy umbrella toxins against their competitors
News & Views 17 APR 24
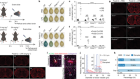
Brain endothelial GSDMD activation mediates inflammatory BBB breakdown
Article 17 APR 24
ROS-dependent S-palmitoylation activates cleaved and intact gasdermin D
Article 10 APR 24
Postdoctoral Associate
Houston, Texas (US)
Baylor College of Medicine (BCM)
Postdoctoral Research Fellow
Description Applications are invited for a postdoctoral fellow position at the Lunenfeld-Tanenbaum Research Institute, Sinai Health, to participate...
Toronto (City), Ontario (CA)
Sinai Health
Postdoctoral Research Associate - Surgery
Memphis, Tennessee
St. Jude Children's Research Hospital (St. Jude)
Open Rank Faculty Position in Biochemistry and Molecular Genetics
The Department of Biochemistry & Molecular Genetics (www.virginia.edu/bmg) and the University of Virginia Cancer Center
Charlottesville, Virginia
Biochemistry & Molecular Genetics
Postdoctoral Position - Synthetic Cell/Living Cell Spheroids for Interactive Biomaterials
Co-assembly of artificial cells and living cells to make co-spheroid structures and study their interactive behavior for biomaterials applications.
Mainz, Rheinland-Pfalz (DE)
University of Mainz
Sign up for the Nature Briefing newsletter — what matters in science, free to your inbox daily.
Quick links
- Explore articles by subject
- Guide to authors
- Editorial policies

An official website of the United States government
The .gov means it’s official. Federal government websites often end in .gov or .mil. Before sharing sensitive information, make sure you’re on a federal government site.
The site is secure. The https:// ensures that you are connecting to the official website and that any information you provide is encrypted and transmitted securely.
- Publications
- Account settings
Preview improvements coming to the PMC website in October 2024. Learn More or Try it out now .
- Advanced Search
- Journal List
- Stem Cell Res Ther

Current progress in stem cell therapy for type 1 diabetes mellitus
Key Laboratory of Longevity and Ageing-Related Disease of Chinese Ministry of Education, Center for Translational Medicine and School of Preclinical Medicine, Guangxi Medical University, Nanning, 530021 Guangxi China
Chunlin Zou
Associated data.
Not applicable.
Type 1 diabetes mellitus (T1DM) is the most common chronic autoimmune disease in young patients and is characterized by the loss of pancreatic β cells; as a result, the body becomes insulin deficient and hyperglycemic. Administration or injection of exogenous insulin cannot mimic the endogenous insulin secreted by a healthy pancreas. Pancreas and islet transplantation have emerged as promising treatments for reconstructing the normal regulation of blood glucose in T1DM patients. However, a critical shortage of pancreases and islets derived from human organ donors, complications associated with transplantations, high cost, and limited procedural availability remain bottlenecks in the widespread application of these strategies. Attempts have been directed to accommodate the increasing population of patients with T1DM. Stem cell therapy holds great potential for curing patients with T1DM. With the advent of research on stem cell therapy for various diseases, breakthroughs in stem cell-based therapy for T1DM have been reported. However, many unsolved issues need to be addressed before stem cell therapy will be clinically feasible for diabetic patients. In this review, we discuss the current research advances in strategies to obtain insulin-producing cells (IPCs) from different precursor cells and in stem cell-based therapies for diabetes.
Introduction
Diabetes mellitus (DM) is a group of chronic metabolic disorders characterized by hyperglycemia due to insufficient secretion of insulin or insulin resistance. DM is mainly divided into four categories: type 1 diabetes mellitus (T1DM), type 2 diabetes mellitus (T2DM), gestational diabetes, and monogenic diabetes. Patients with T1DM need daily insulin injections because of the absolute insufficiency of endogenous insulin caused by autoimmune destruction of pancreatic β cells. Thus, type 1 diabetes is also known as insulin-dependent DM. Patients with type 2 diabetes may need exogenous insulin injections when oral medications cannot properly control the blood glucose levels. Diabetes without proper treatment can cause many complications. Acute complications include hypoglycemia, diabetic ketoacidosis, or hyperosmolar nonketotic coma (HHNC). Long-term complications include cardiovascular disease, diabetic nephropathy, and diabetic retinopathy [ 1 ]. Although hyperglycemia can be ameliorated by drugs or exogenous insulin administration, these treatments cannot provide physiological regulation of blood glucose. Therefore, the ideal treatment for diabetes should restore both insulin production and insulin secretion regulation by glucose in patients (Fig. 1 ).

Attempts to cure T1DM. The discovery of insulin has enhanced the life span of T1DM patients, and successes in islet/pancreas transplantation have provided direct evidence for the feasibility of reestablishing β cells in vivo to treat T1DM. However, the restriction of a pancreas shortage has driven scientists to generate IPCs, and even whole pancreas, in vitro from hESCs, iPSCs, and adult stem cells. Studies focusing on the immune mechanism of T/B cell destruction in T1DM have made breakthroughs. Gene therapy has shown great promise as a potential therapeutic to treat T1DM, although its safety still needs to be confirmed in humans
Clinical pancreas or islet transplantation has been considered a feasible treatment option for T1DM patients with poor glycemic control. Dr. Richard Lillehei performed the first pancreas transplantation in 1966 [ 2 ]. Up until 2015, more than 50,000 patients (> 29,000 in the USA and > 19,000 elsewhere) worldwide had received pancreas transplantations according to the International Pancreas Transplant Registry (IPTR) [ 3 ]. Islet cell transplantation was first performed in 1974. However, efforts toward routine islet cell transplantation as a means for reversing type 1 diabetes have been hampered by limited islet availability and immune rejection. In 2000, Shapiro et al. reported that seven consecutive patients with type 1 diabetes attained sustained insulin independence after treatment with glucocorticoid-free immunosuppression combined with the infusion of adequate islet mass. Moreover, tight glycemic control and correction of glycated hemoglobin levels were observed in all seven patients. This treatment became known as the Edmonton protocol [ 4 ]. Over the past two decades, continuous improvements in islet isolation and immunosuppression have increased the efficiency of pancreatic islet transplant, and approximately 60% of patients with T1DM have achieved insulin independence 5 years after islet transplantation [ 3 , 5 – 8 ].
However, the worldwide shortage of pancreas donors in clinical islet transplantation remains a major challenge. Intensive studies have been conducted for the generation of IPCs or islet organoids in vitro since human pluripotent stem cells (hPSCs) have been anticipated for application in regenerative medicine. The sources for the generation of IPCs or islet organoids in vitro mainly include hPSCs (human embryonic stem cells (hESCs) and human induced pluripotent stem cells (hiPSCs)), adult stem cells, and differentiated cells from mature tissues that can be transdifferentiated into IPCs. Current strategies for generating IPCs are mainly based on approaches that mimic normal pancreas development. The obtained IPCs are supposed to express specific biological markers of normal β cells that identify a terminal differentiation status, such as MAFA (a basic leucine zipper transcription factor expressed in mature β cells and absent in pancreatic progenitors and other cell types), NEUROD1 (downstream factor of NGN3 expressed in most pancreatic endocrine cells, including β cells), and PDX1/NKX 6.1 (restricted coexpression in β cells), as well as key functional features of adult β cells, including glucose-stimulated insulin secretion (GSIS) and C-peptide secretion [ 9 – 14 ]. In addition, after implantation into DM patients or immunodeficient diabetic animals, these in vitro-generated IPCs or islet organoids should respond to changing blood glucose and produce sufficient insulin and finally reverse hyperglycemia.
In the last two decades, many protocols have been successfully designed for the generation of IPCs or islet organoids in vitro. In this review, we summarized the research progress in the generation of IPCs and islet organoids from hPSCs and adult stem cells and the new technological advances in stem cell-based therapy for T1DM.
Generating IPCs from embryonic stem cells (ESCs) and induced pluripotent stem cells (iPSCs)
ESCs are pluripotent cells isolated from the inner cell mass of a blastocyst, the early mammalian embryo that implants into the uterus. ESCs show the characteristics of infinite proliferative capacity and self-renewal and are able to differentiate into multiple types of adult cells in vitro [ 15 ]. iPSCs, which are reprogrammed from somatic cells, hold a similar capacity to proliferate and differentiate like ESCs. Hence, hPSCs provide a promising platform to produce in vitro insulin-secreting cells. Ethical issues in the applications of ESCs are still controversial due to their origins. In contrast, iPSCs are derived from adult somatic cells that have been reprogrammed back into an embryonic-like pluripotent state using Yamanaka factors [ 16 , 17 ]. During the last two decades, numerous methods to generate IPCs from hPSCs have been reported [ 9 – 12 , 18 – 22 ].
Ordinarily, the schemes for the generation of functional IPCs from hPSCs were based on imitating the in vivo development of the embryonic pancreas (Fig. 2 ). The pivotal stages of embryonic pancreas development include the development of the definitive endoderm (DE), primitive gut tube (PGT), pancreatic progenitor (PP), endocrine progenitor (EP), and hormone-expressing endocrine cells. By adding diverse cytokines (e.g., epidermal growth factor, bFGF) and signaling modulators (e.g., bone morphogenetic proteins, γ-secretase inhibitors) to each stage to activate or inhibit specific signaling pathways (e.g., Notch, Wnt) involved in the generation of adult β cells, the hPSC cell fate is manipulated into the β cell phenotype [ 18 , 20 , 23 ].

Generation of insulin-producing β cells from hPSCs. Schematic illustration of the differentiation protocol for generating insulin-producing β cells from hPSCs by mimicking the in vivo development of the embryonic pancreas. The key molecules of all key developmental stages of pancreatic islet β cells are illustrated
D’Amour et al. set up the first stepwise protocol to produce endocrine hormone-expressing cells that were able to synthesize and release multiple hormones from hESCs. However, at the final stage, the average percentage of insulin-positive cells in differentiated hES cell cultures was only 7.3%. Furthermore, these polyhormonal cells failed to respond to a high-glucose stimulus [ 18 ]. It is known that the fetal pancreas also possesses these characteristics, and previous studies demonstrated that fetal human pancreatic tissues could develop functionally after transplantation into animals [ 24 – 27 ]. Thus, the authors chose to determine whether these immature β cells derived from hESCs could mature into functional β cells under an in vivo environment. They generated pancreatic endoderm cells (similar to fetal 6- to 9-week pancreatic tissue) using an optimized protocol and then transplanted them into immunodeficient mice. The pancreatic endoderm cells successfully differentiated and matured into β-like cells in response to both fasting-induced hypoglycemia and glucose challenge and maintained normal glucose homeostasis for 3 months [ 28 ].
Similarly, the generation of IPCs from iPSCs is based on consecutive regulation of specific signaling pathways involved in pancreas development. Tateishi et al. first demonstrated that skin fibroblast-derived iPSCs were capable of producing islet-like clusters (ILCs) in vitro by mimicking the in vivo development of the pancreas. However, under high glucose stimulation (40 mM), the amount of C-peptide secreted by iPSC-derived ILCs and ESC-derived ILCs was only 0.3 ng/μg DNA and 0.15 ng/μg DNA, respectively [ 29 ].
Although the above studies have confirmed that hESCs and hiPSCs have the potential to differentiate into IPCs, this differentiation is done only cautiously owing to the low differentiation efficiency of protocols and the polyhormonal features of these β-like cells.
One of the breakthroughs comes from Rezania et al. in 2014, and the authors reported a more detailed protocol and generated mature and functional IPCs from hPSCs that were comparable to human β cells. The differentiation protocol was divided into 7 sequential stages, including definitive endoderm (stage 1), primitive gut hub (stage 2), posterior foregut (stage 3), pancreatic endoderm (stage 4), pancreatic endocrine precursors (stage 5), immature β cells (stage 6), and maturing β cells (stage 7). The obtained cells expressed key markers of mature β cells, such as MAFA, PDX1/NKX6.1, and INS, and showed functional similarities to human islets after transplantation in vivo. These β-like cells rapidly reversed hyperglycemia in STZ-diabetic mice by secreting C-peptide and insulin [ 20 ]. Nevertheless, the S7 (stage 7) cells were not equivalent to mature human β cells. S7 cells exhibited a very small and blunt response to high glucose stimulation, which differs from that of mature islet β cells. Moreover, a scalable suspension-based culture system developed by Paliuca et al. showed the possibility of generating large-scale stem cell-derived β cells (SC-β) [ 9 ]. Expression of NGN3 marks the initiation of endocrine differentiation. Previous studies have confirmed that inhibition of the Notch signaling pathway using γ secretase inhibitors or BMP inhibitors is essential for the induction of NGN3, followed by the addition of fibroblast growth factor 10 and keratinocyte growth factor (KGF), resulting in the robust generation of PDX1 + pancreatic progenitors and an increase in insulin expression in hPSC-derived progeny [ 9 , 20 ]. However, Russ et al. demonstrated that the use of BMP inhibitors promoted the precocious induction of endocrine differentiation in PDX1 + pancreatic progenitors and that omitting addition at pancreatic specification could successfully reduce the formation of polyhormonal cells. Subsequent exposure to retinoic acid and epidermal growth factors (EGF)/KGF cocktail efficiently induced the formation of PDX1 + /NKX6.1 + progenitor cells that differentiated into IPCs in vitro [ 10 ]. Recently, Yabe et al. reported that the addition of the selective glycogen synthase-kinase-3 β (GSK-3β) inhibitor (a substitute for Wnt3a; regarded as a key molecule for definitive endodermal induction from hPSCs) during definitive endodermal induction significantly decreased the death rate of endodermal cells [ 12 , 18 , 30 ]; further, spheroid formation of postendocrine progenitor cells rather than monolayer formation was crucial for generating IPCs from hiPSCs, which may be explained by the unique architecture of adult islets.
Among the above studies, the obtained cell population contains an average of 45% β cells, and the phenotypes of the remaining cells were unclarified. Identification of cell types that formed during differentiation is particularly important to improve the differentiated proportion of β cells. In a recent study, single-cell RNA sequencing in hPSCs undergoing in vitro β cell differentiation mapped a comprehensive description of cell production during stem-to-β cell differentiation [ 31 ]. Four distinct cell populations were isolated and identified from stem cell-derived islets, including SC-β cells, α-like polyhormonal cells, nonendocrine cells, and stem cell-derived enterochromaffin (SC-EC) cells. An in vitro study confirmed that α-like polyhormonal cells were transient toward SC-α cells and that nonendocrine cells were capable of generating exocrine cells (pancreatic acinar, mesenchymal and ductal cells). Additionally, CD49a was characterized as a surface marker of SC-β cells but not of adult islet β cells. Furthermore, SC-β cells could be purified up to 80% from SC islets using a scalable reaggregation method and magnetic sorting.
As patient-derived hiPSCs have been shown to provide tremendous advantages for studying the pathogenesis and pathophysiology of disease in vitro, studies on producing iPSCs from diabetic patients have generated great interest. Patient-specific iPSCs can overcome current obstacles in stem cell therapy, such as immune rejection and immune mismatch, and provide a platform to establish a personalized disease model to investigate pathogenic mechanisms and seek therapeutic methods for the disease. Maehr et al. successfully generated hiPSCs from skin fibroblasts of patients with T1DM (T1DM-specific iPSCs, DiPSCs). These DiPSCs resembled ESCs in the global gene expression profile and were capable of differentiating into pancreatic cell lineages, paving the path of generating T1DM SC-β cells and making autologous stem cell-derived pancreatic progeny transplantation for T1DM possible [ 32 ]. In 2015, Millman et al. confirmed that SC-β cells derived from DiPSCs functionally resembled adult islet β cells both in vivo and in vitro. GSIS tests showed that under high glucose stimulation (20 mM incubation for 30 min), T1DM and nondiabetic (ND) SC-β cells secreted 2.0 ± 0.4 and 1.9 ± 0.3 mIU of human insulin per 10 3 cells, respectively, and both of these cells functioned similarly to adult primary islets in a previous study. After transplantation into ND immunodeficient mice, the engraft function was evaluated by serum human insulin before and 30 min after an injection of glucose. At the early time point (2 weeks after transplantation), most engrafts responded to glucose and released more insulin after glucose injection, and the ratio of insulin secretion after glucose stimulation averaged 1.4 and 1.5 for T1DM and ND SC-β cells, respectively. The effects of these engrafts on insulin secretion were observed for several months. Of note, compared to the early time point, after 12–16 weeks, the human insulin content increased approximately 1.5 times after glucose stimulation [ 33 ]. It should be acknowledged that diversities exist among T1DM patients, and a larger number of specific stem cell lines from T1DM need to be developed for future clinical use. Although DiPSCs are an alternative source for cell replacement therapy for diabetes, some T1DM-specific stem cell lines have shown low efficiency in generating PDX1 + pancreatic progenitors [ 34 ]. Evaluated by flow cytometry, the number of IPCs derived from ND iPSCs (25–50.5%) was comparable to that of the β cells found in human primary islets, whereas the number of IPCs differentiated from T1DM iPSC lines was much lower (15.9%) [ 35 , 36 ]. Upon a strict differentiation protocol, pancreatic progenitors derived from T1DM iPSCs showed lower expression of PDX1 than ND iPSCs at a specific differentiation stage. Epigenetic changes resulting from dysmetabolism in T1DM might be responsible for the poor yield of β cells from T1DM iPSCs. Transient demethylation treatment of DE cells rescued the expression of PDX1 by inhibiting methyl group deposition on the cytosine residues of DNA and led to the differentiation of DE cells into IPCs [ 36 ]. The effect of demethylation on IPC differentiation has been shown to promote pancreatic progenitor induction rather than DE induction [ 37 ].
Generating pancreatic progenitors from ESCs and iPSCs
Pancreatic progenitors that coexpress specific markers indispensable for inducing a β-cell fate are a crucial cell state of differentiating hPSCs into β cells in vitro. Pancreatic and duodenal homeobox 1 (PDX1) transcription factor and NK6 homeobox transcription factor-related locus 1 (NKX6.1) have been considered to be the regulatory factors of differentiating DE into pancreatic progenitors [ 38 ]. Notably, high coexpression of PDX1 and NKX6.1 in pancreatic progenitors is essential for the efficient generation of mature and functional β cells [ 39 , 40 ].
Of note, the efficiency and safety of pancreatic progenitors that coexpress PDX1 and NKX6.1 for T1DM treatment are currently being evaluated in clinical trials by ViaCyte Company. Thus, elevating the production of hPSC-derived β cells, optimizing the in vitro differentiation protocols in multiple aspects, and generating a high population of PDX1 + /NKX6.1 + pancreatic progenitors are needed to accelerate the clinical trial. Multiple studies have been carried out to determine the appropriate cocktail of cytokines to mimic in vivo development [ 41 – 43 ]. Recently, Nostro et al. demonstrated that the combination of EGF and nicotinamide induced a higher production of NKX6.1 + pancreatic progenitors in adherent culture [ 44 ]. Importantly, the authors focused on the temporal window of foregut differentiation into the pancreatic endoderm and confirmed that the size of the NKX6.1 + population decreased with extended duration. Although previous studies have shown that the maintenance of cellular aggregation during the differentiation process could significantly elevate the efficiency of pancreatic progenitors [ 10 , 45 , 46 ], the impact of culture condition changes that affect the physical environment of cells on pancreatic progenitor differentiation is still less studied. Memon et al. showed that the generation of PDX1 + /NKX6.1 + pancreatic progenitors could be dramatically induced after dissociating and replating pancreatic endodermal cells at half density in monolayer culture [ 47 ]. Intriguingly, a novel NKX6.1 + /PDX1 − cell population that holds the potential to generate functional β cells was discovered, and the cell type was confirmed to be a new type of pancreatic progenitor cell by the same team [ 48 ].
Another important issue that needs to be resolved before hPSC-derived pancreatic progenitors can be used in the clinic is how the recipient’s in vivo environment affects the maturation and differentiation of these undifferentiated cells. Although many studies have highlighted the importance of the in vivo environment in promoting islet cell differentiation, the system mechanism regulating the response of the transplanted cells to the in vivo environment has not been well studied [ 9 , 20 , 21 ]. Most recently, Legøy et al. confirmed that short-term exposure of encapsulated pancreatic progenitors to an in vivo environment was beneficial for cell fate determination, as revealed by increased islet proteome characteristics [ 49 ]. These effects could be partially mediated by the levels of hepatocyte nuclear factor 1-α (HNF1A) and hepatocyte nuclear factor 4-α (HNF4A) in recipients.
Generating islet organoids/islets from ESCs and iPSCs
The pancreatic islet of Langerhans is comprised of α, β, δ, ε, and pancreatic polypeptide cells [ 46 , 50 ]. Many studies have highlighted the importance of reciprocal coordination and complementary interactions of different types of islet cells for glucose hemostasis [ 51 – 54 ]. Thus, it may be beneficial for producing whole islets or islet organoids rather than differentiating cells into a specific type.
Organoids are defined as 3D cultures maintained in vitro that can be generated from adult tissues or hPSCs and recapitulate the in vivo morphologies, cellular architecture and organ-specific functionality of the original tissue. Kim et al. developed islet-like organoids from hPSCs that showed a glucose response in vitro and in vivo [ 55 ]. Endocrine cells (ECs) were generated from hPSCs using a multistep protocol and expressed pancreatic hormones. Notably, dissociated ECs spontaneously formed islet-like spheroids, referred to as endocrine cell clusters (ECCs), under optimal 3D culture conditions in 24 h. The diameter of the ECCs was approximately 50–150 μm and contained 5 × 10 4 cells. ECCs consisted of several types of islet endocrine cells, apart from α cells, indicating that ECCs derived from hPSCs are partially similar to human adult islets. After high glucose stimulation (27.5 mM) for 1 h, ECCs showed increases in both insulin and C-peptide secretion, from 1.01 ± 0.22% up to 2.6 ± 0.21% and from 159.6 ± 20.01 pmol/L up to 336.3 ± 29.21 pmol/L, respectively. Additionally, ECCs exhibited intracellular Ca 2+ oscillation under a high glucose stimulus. Furthermore, a major breakthrough was that after ECCs were implanted into STZ-induced diabetic mice, normoglycemia was rapidly achieved within 3 days. In previous studies, transplanted hPSC-derived ECs took a long period (over 40 days) to normalize the glucose level in diabetic mice [ 9 , 10 , 20 , 28 ]. Therefore, this study suggested that it was promising to generate functional islet-like organoids from hPSCs and provided an alternative cell source for treating diabetes. Soon after that, based on a biomimetic 3D scaffold, islet organoids were successfully generated from hESCs [ 56 ]. The organoids contained all types of pancreatic cells (α, β, δ, and pancreatic polypeptide cells), specific markers of mature β cells as well as insulin secretory granules, which were characterized by a round electron-dense crystalline core surrounded by a distinctive large, clear halo. Insulin granules have been reported as an indication of mature β cells and a key participant in glucose homeostasis [ 36 , 57 ]. Generally, insulin granules in adult β cells were differentiated according to the shape and density of the core. Through transmission electron microscopy, insulin granules generally possess a characteristic “halo,” which is a product of glutaraldehyde fixation that does not exist in other endocrine granules. Many studies have reported remarkable insulin granules during the differentiation of hPSCs into IPCs [ 9 , 20 ]. Glucose loading experiments demonstrated that islet organoids exhibited a sharp increase in insulin secretion under high glucose conditions. Under the same glucose stimulation conditions (exposure from 5.5 mM to 25 mM), the 3D-induced cells had an insulin content that increased by seven-fold, whereas the 2D-induced cells had an insulin content that increased by 3.7-fold. These results suggested that 3D-induced IPCs are more sensitive to glucose stimulation due to their elevated maturity.
Fundamental studies of islet development during embryogenesis will promote optimization of protocols for differentiating hPSCs into 3D islet clusters or islet organoids. The traditional model of islet development is based on epithelial-mesenchymal transition (EMT) during the differentiation of pancreatic progenitors. However, this hypothesis was recently challenged by a study in which the dynamic changes in transcripts involved in islet formation were mapped [ 46 ]. Sharon et al. reported that along with EP differentiation, they maintained intact cell-to-cell adhesion and formed bud-like islet precursors (defined as peninsula-like structures) rather than undergoing EMT. Further in vitro generation of SC-β cells showed that the maintenance of cell adhesion could efficiently induce hESCs into peninsula-like structures. Importantly, these peninsula-like clusters could generate INS + and GCG + monohormonal cells after transplantation into SCID mice. This study provides a new framework for understanding islet embryogenesis and offers novel ideas to optimize the current protocols for the differentiation of SC-β cells.
Generating interspecific pancreatic chimeras from pancreatic stem cells (PSCs)
Interspecific chimeras, defined as organisms with cells originating from at least two different species, are able to produce organs completely consisting of donor-origin cells. Thus, human-animal chimeras have great potential for providing immune-compatible patient-specific human organs for transplantation.
In 2010, Kobayashi et al. successfully generated a functional rat pancreas in PDX1 −/− (pancreatogenesis knockout) mice via interspecies blastocyst complementation [ 58 ]. The rat iPSC-derived pancreas (rat M pancreas) in PDX1 −/− mice showed both exocrine and endocrine characteristics and expressed several pancreatic enzymes and hormones. In addition, outcomes from glucose tolerance testing (GTT) in adulthood indicated that endogenous insulin secretion was increased under high blood glucose, and glucose homeostasis was preserved. Recently, the same group reported the reverse experiment; mouse PSCs were injected into PDX1 −/− rat blastocysts to generate a pancreas (mouse R pancreas) the size of a rat pancreas with pancreatic cells primarily originating from mouse PSCs [ 59 ]. Most importantly, the isolated islets from the mouse R pancreas were subsequently injected into STZ-induced diabetic mice, and functional glucose-induced insulin secretion was successfully established in recipients for over 1 year. These data strongly supported the hypothesis that donor PSC-derived organs could be generated in a xenogeneic environment and provided the theoretical possibility of applying donor PSC-derived islets generated by animal-human interspecific blastocyst complementation in clinical trials. It is worth noting that rat M pancreases were the size of a rat pancreas, rather than the size of a mouse pancreas or an intermediate size, whereas mouse R pancreases were the size of a mouse pancreas. Thus, to adapt interspecific blastocyst complementation for patients, it seems necessary to generate organs in animals that are closer to humans in both size and evolutionary distance, such as sheep, pigs, and nonhuman primates (NHPs). Exogenic pancreases have been generated in vivo in transgenic cloned pigs by blastocyst complementation [ 60 ]. In this study, donor morula blastomeres derived from female cloned embryos were injected into the morula of male pancreatogenesis-disabled fetuses, and morphologically and functionally normal donor-derived pancreases were formed in adult chimeric pigs. Furthermore, PDX1 −/− sheep generated using CRISPR/Cas9 have been reported and can potentially serve as a host for interspecies organ generation [ 61 ]. However, blastocyst complementation has failed to generate chimeras in NHPs [ 62 ].
Differentiation of adult stem cells into IPCs
The search for adult pancreatic stem cells.
The adult pancreas consists of two unique parts: the exocrine pancreas and the endocrine pancreas, with unique morphology and function, respectively. The pancreas arises from two separate primordia along the dorsal and ventral surfaces of the posterior foregut. Lineage-tracing studies have demonstrated that all of the mature pancreatic cells were developed from PDX1 + /PTF1A + progenitor cells [ 63 , 64 ]. However, if there are detectable pancreatic stem cells in adult animal and human pancreases, how these cells participate in the regeneration of β cells is still under debate. The hypothesis was initially supported by histological observation of neogenesis occurring in adult rodent pancreatic ducts after pancreatic duct ligation (PDL) [ 65 ]. However, genetic lineage-tracing studies indicated that there was no contribution to endocrine regeneration during the adult life or after injury, and the major mechanism was enhanced replication by only preexisting β cells [ 63 , 66 , 67 ]. In 2007, supporting evidence comes from a study by Xu et al., in which NGN3 + (the earliest islet cell-specific transcription factor) endocrine precursors appeared in the ductal lining after PDL in mice and gave rise to all types of islet cells, including glucose-responsive β cells [ 68 ]. Additionally, increased proliferation and ectopic NGN3 + pancreatic progenitors were reported in experiments of α-to-β-cell reprogramming [ 69 , 70 ]. In conclusion, whether adult pancreatic stem cells exist in adulthood is unclear. Recent events in single-cell RNA sequencing are promising for mapping dynamic gene expression changes during the adult lifespan or after injury in animal and human pancreases, for constructing differentiation trajectories of pancreas/islet cells and for illustrating the mechanisms involved in β cell regeneration.
Pancreatic duct-derived stem cells
Theoretically, pancreatic duct epithelial cells possess a promising capacity for β cell generation because both originate from the same embryonic precursor [ 46 , 71 ]. Budding of β cells or new islets generated from ductal epithelium occurs during pancreatic regeneration in adults and has been reported [ 72 , 73 ]. Since then, studies have been designed to reprogram pancreatic ductal cells into β cells. Ramiya et al. isolated pancreatic ductal epithelial cells from prediabetic adult nonobese diabetic (NOD) mice, cultured them in vitro, and ensued the formation of ILCs that contained α, β, and δ cells. Subsequently, the blood glucose level of diabetic NOD mice was decreased from 400 to 180–220 mg/dl in 7 days [ 74 ]. Moreover, Bonner-Weir et al. demonstrated that the pancreatic ductal epithelium could expand and further differentiate into functional islet tissues in a Matrigel-based 3D culture system in vitro [ 75 ]. Further studies demonstrated that CK19 + nonendocrine pancreatic epithelial cells (NEPECs) can be differentiated into β cells in vitro [ 76 ].
Over the past two decades, attempts have been directed toward optimizing the protocols for generating IPCs from pancreas duct-derived stem cells. Since CA19-9 and CD133 were identified as specific membrane proteins of pancreas duct-derived stem cells, it became easier to purify these cells from the adult human pancreas [ 77 , 78 ]. It has been demonstrated that diverse growth factors (e.g., bFGF, EGF, and KGF) benefit the proliferation and differentiation of human pancreatic duct-derived stem cells [ 74 , 79 ]. Generally, epithelial cells show limited mitotic activity in vitro. Corritore et al. developed a differentiation protocol in which isolated human pancreatic duct cells from the pancreas were forced to undergo EMT to achieve a phenotypic change and allow them to extensively proliferate. After proliferation of these cells in vitro, pancreatic duct-derived cells differentiated into IPCs with a large array of specific marker expression and insulin secretion [ 78 ]. More recently, Zhang et al. reported that diabetic mice continuously administered gastrin and EGFs had accelerated transdifferentiation of SOX9 + duct cells into IPCs and consequently maintained blood glucose homeostasis [ 80 ].
Nestin-positive mesenchymal stem cells from islets
Nestin is an intermediate filament protein that is specifically expressed in neuronal and muscle precursor cells [ 81 , 82 ]. Recent studies have indicated that nestin-positive (nestin + ) cells resided in pancreatic islets and could differentiate into IPCs and islet-like cell clusters (Fig. 3 ), and now, nestin has been accepted as a critical pancreatic progenitor marker [ 83 , 84 ]. Zulewski et al. first demonstrated the existence of a distinct cell population within islets isolated from the human pancreas that express nestin, termed nestin-positive islet-derived progenitor cells (NIPs). These NIPs displayed features of stem cells and were able to generate cells with either pancreatic exocrine or endocrine phenotypes in vitro. Most importantly, the terminally differentiated cells were capable of secreting pancreatic hormones, such as insulin and glucagon [ 85 ]. Another study performed by the same group reported that NIPs also showed characteristics of bone marrow side population (SP) stem cells due to their coexpression of the ATP-binding cassette transporter ABCG2, which has been previously demonstrated to be a major component of the SP phenotype [ 85 – 87 ]. This was further supported by a study showing that NIPs isolated from a human fetal pancreas expressed ABCG2 and nestin [ 88 ]. Moreover, CD44, CD90, and CD147, which represent the phenotypes of bone marrow-derived mesenchymal stem cells, were also detected on NIPs. These data strongly indicated that NIPs have a high potential to become an alternative cell source for producing IPCs and islets in vitro. Huang et al. isolated and cultured NIPs from a human fetal pancreas. In this study, NIPs formed islet-like cell clusters (ICCs) in confluent cultures. Moreover, differentiation of ICCs from NIPs results in increased pancreatic islet-specific gene expression, along with a concomitant downregulation of ABCG2 and nestin. Additionally, the transplantation of ICCs reversed hyperglycemia in diabetic NOD-SCID mice [ 89 ].

Generation of IPCs from adult stem cells. Adult pancreatic stem cells may be a potential source of IPCs. Functional IPCs have been generated from pancreatic ductal cells and NIPs isolated from adult islets. During embryogenesis, the liver and pancreas arise from common endoderm progenitors. Liver cells can transdifferentiate into IPCs by ectopic expression of pancreatic transcription factors. Additionally, a high pluripotent cell population termed HLSCs can also produce IPCs in vitro. Bone marrow-derived stem cells show the capacity to generate insulin cell clusters
The studies mentioned above about NIPs are based on rodent models. Nonhuman primate models often serve as an important bridge from laboratory research to clinical application; thus, generating pancreatic stem cells/progenitor cells from NHPs has led to great interest. Our previous study indicated that pancreatic progenitor cells existed in the adult pancreases of type 1 diabetic monkeys as well as in the pancreases of normal monkeys. The isolated pancreatic progenitor cells were able to proliferate in vitro and form ICCs in differentiation media. Furthermore, glucose-induced insulin and C-peptide secretion from the ICCs suggested that the ICCs functionally resembled primary islets [ 90 ]. In view of pathogenetic differences between STZ-induced diabetic monkeys and patients with T1DM, it still needs to be clarified whether NIPs also reside in T1DM patients.
Differentiation of bone marrow-derived stem cells (BMDSCs)
Several studies have reported that BMDSCs have the ability to differentiate into IPCs. Tang et al. reported that BMDSCs could spontaneously differentiate and form ICCs when continuously cultured with high glucose concentrations. The ICCs expressed multiple pancreatic lineage genes, including INS, GLUT2, glucose kinase, islet amyloid polypeptide, nestin, PDX-1, and PAX6, with β cell development. Moreover, ICCs could respond to glucose stimulation and release insulin and C-peptide in vitro, and following implantation into diabetic mice, hyperglycemia was reversed [ 91 ]. Since then, numerous studies have demonstrated the generation of IPCs from human and rat bone marrow stem cells (Fig. (Fig.3). 3 ). However, the efficacy of BMDSC differentiation is low and highly variable with the current protocols. In particular, the quantity of insulin secreted by these cells was far from that secreted by adult β cells. Gabr and colleagues tested the efficiency of three differentiation protocols using immunolabeling, and the proportion of generated IPCs was modest (≈ 3%) in all protocols [ 92 ]. The expression of pancreatic-associated genes in generated IPCs was quite low compared to the expression in human islets. Optimizing differentiation protocols to upregulate the expression of specific genes by determining optimal molecules and culture conditions is crucial. Extracellular matrix proteins play a vital role in cell differentiation and proliferation. Laminin, one of the pancreatic extracellular matrices, has been confirmed to enhance the expression of insulin and promote the formation of ICCs from BMDSCs, whereas collagen type IV affects the expression of NEUROD1 and GCG [ 93 ]. Generally, differentiation of BMDSCs into IPCs is performed on nonadherent polymer surfaces and hydrogels. A recent study reported that 3D culture of BMDSCs on agar (a hydrogel-forming polysaccharide widely used in biomedical research) for 7 days followed by 2D culture of formed cellular clusters in high glucose media could enhance the production of IPCs from BMDSCs [ 94 ]. IPCs expressed INS genes at a 2215.3 ± 120.8-fold higher level than BMDSCs, whereas this fold change in previous studies was 1.2–2000-fold.
Differentiation of liver cells
The liver and pancreas originate from appendages of the upper primitive foregut endoderm. Later, separation of the liver and pancreas during organogenesis left both tissues with multipotent cells capable of generating both hepatic and pancreatic cell lineages. The common embryonic origin of the liver and pancreas raises the intriguing speculation that it may be possible to convert liver cells to pancreatic ECs (Fig. (Fig.3). 3 ). Several studies have demonstrated that adult or fetal liver cells and biliary epithelial cells are capable of reprogramming into IPCs by inducing the expression of endocrine pancreatic-specific transcription factors [ 95 – 98 ]. The in vivo data showed that these hepatic cell-derived IPCs could ameliorate hyperglycemia upon implantation into diabetic mice. However, the efficiency of liver-to-pancreas reprogramming is still low, and the obtained IPCs are likely immature β-like cells. In addition, Herrera et al. isolated and characterized a population of human liver stem cells (HLSCs). HLSCs express both mesenchymal stromal cells (MSCs) and immature hepatocyte markers. In addition, HLSCs expressing nestin and vimentin are capable of differentiating into multiple cell lineages, including epithelial, endothelial, osteogenic, and islet-like structure (ILS) cells [ 99 ]. Later, Navarro-Tableros et al. confirmed that HLS-ILS cells expressed β cell transcription factors, such as NKX6.1, NKX6.3, and MAFA, and could respond to glucose loading by releasing C-peptide. Hyperglycemia was rapidly reversed in diabetic SCID mice after implantation [ 100 ]. These data suggest that HLSCs could be a novel potential resource for stem cell-based therapy for diabetes.
Encapsulation technique for stem cell therapy for T1DM
The encapsulation technique is based on a matrix that prevents immune cells, cytokines, and antibodies from reacting to grafts while allowing nutrient, oxygen, and signaling molecule diffusion. An appropriate encapsulation device is especially crucial for T1DM to prevent an autoimmune reaction against transplanted hPSC-derived pancreatic progeny, including allogenic grafts. Criteria to evaluate an encapsulation device should take many variables into consideration, including the biocompatibility, stability and permselectivity of the membrane, interaction with the bloodstream, availability of nutrients and oxygen, among others [ 101 – 103 ]. Studies have been performed to detect optimal materials to improve these properties and have mainly been developed for pancreatic islet transplantation.
Alginate, a scaffolding polysaccharide produced by brown seaweeds, has been widely employed by virtue of its biocompatibility [ 102 , 104 , 105 ]. Alginates are linear unbranched polymers containing β-(1 → 4)-linked d -mannuronic acid (M) and α-(1 → 4)-linked l -guluronic acid (G) residues and possess eminent gel-forming properties in the presence of polyvalent cations, such as Ca 2+ and Ba 2+ [ 103 , 106 – 108 ]. Earlier studies have confirmed that compared to nonencapsulated islets, encapsulated islets have significantly improved survival, long-term biocompatibility and function with the use of purified alginate [ 109 – 112 ]. Additionally, specific modifications to alginates trigger great interest, as they could circumvent the local immune response after transplantation of an allo- or xenograft. The incorporation of the chemokine CXCL2 with alginate microcapsules prevented allo- or xenoislet transplantation from immune reactions by establishing sustained local immune isolation [ 113 ]. Most recently, the same team confirmed that these modifications on alginates could also efficiently prolong the survival and function of hPSC-derived β cells and achieve long-term immunoprotection in immunocompetent mice with T1DM without systemic immunosuppression [ 114 ]. Of note, CXCL2 enhanced the GSIS activity of β cells, thus making it a crucial biomaterial to study for stem cell-based therapy for T1DM.
ViaCyte, leading the first and only islet cell replacement therapies derived from stem cells for diabetes, is testing for the safety and efficacy of its encapsulation devices PEC-Encap and PEC-Direct in clinical trials. The PEC-Encap is designed to fully contain hPSC-derived pancreatic progenitors in a semipermeable pouch so that vital nutrients and proteins can travel between the cells inside the device and the blood vessels, which grow along the outside of the device. In the case of PEC-Encap, the implanted cells were completely segregated from the recipients’ immune system. Another device called PEC-Direct allowed blood vessels to enter the device and directly interact with the implanted cells. Thus, immune suppression therapy was necessary for patients who received PEC-Direct, which made it suitable only for people with high-risk type 1 diabetes.
Immune modulation in stem cell therapy for T1DM
Human ESC/iPS-derived β cells have been proposed as a potential β cell replacement source for the treatment of T1DM. However, both the alloimmune and autoimmune responses remain a major problem for the wide application of cell replacement therapies for T1DM. Although massive efforts have been made in the progress of encapsulation technology, the engraftment of transplanted hPSC-derived pancreatic progenitors or β cells still faces challenges. The engraftments will certainly be destroyed by the recipient’s immune system if the encapsulation system is eliminated. Certain modulations of these encapsulated cells to circumvent autoimmune attack seem promising. Human leukocyte antigen (HLA) mismatching is the major molecular mechanism of immune rejection in allo- or xenografts [ 115 ]. Studies have proven that elimination of HLA-A genes by zinc-finger nucleases in hematopoietic stem cells could increase donor compatibility [ 116 , 117 ]. Likewise, knocking out the β2-microglobulin (B2M) gene, which abolishes all HLA class I molecules, or deleting HLA-A and HLA-B biallelically, retained one allele of HLA-C to allow the hPSC grafts to avoid T and NK cell attack [ 118 ]. Other protocols for immunosuppressive effects have been reported, such as targeted overexpression of PDL1-CTLA4Ig in β cells, which efficiently prevented the development of T1DM and allo-islet rejection, in turn promoting the survival of β cell mass [ 119 ]. Therefore, immune modulation strategies for hPSCs could be promising to overcome challenges associated with engraft rejection.
Clinical trials in stem cell therapy for T1DM
In the last few years, controlled clinical trials have been carried out to estimate the efficiency and safety of stem cell therapy for T1DM. It has been demonstrated that MSCs can ameliorate or reverse the manifestation of diabetes in animal models of T1DM. In 2014, Carlsson et al. confirmed that MSC treatment could preserve β cell functions in new-onset T1DM patients. Twenty adult patients (aged 18–40 years) with newly diagnosed (< 3 weeks) T1DM were enrolled and randomized to MSC treatment or to the control group and followed by a 1-year follow-up examination [ 120 ]. At the end of the clinical trial, mixed-meal tolerance tests (MMTTs) revealed that both C-peptide peak values and C-peptide significantly decreased in the treatment group. Of note, MSC treatment side effects were not observed during the follow-up examination. During January 2009 and December 2010, 42 patients aged 18–40 years with a history of T1DM for ≥ 2 years and ≤ 16 years were randomized into either the stem cell transplantation (umbilical cord MSCs in combination with autologous bone marrow mononuclear cells) or standard insulin care treatment groups [ 121 ]. A 1-year follow-up examination indicated that the C-peptide increased from 6.6 to 13.6 pmol/mL/180 min in treated patients, whereas it decreased from 8.4 to 7.7 pmol/mL/180 min in control groups; insulin increased from 1477.8 to 2205.5 mmol/mL/180 min in treated patients; and it decreased from 1517.7 to 1431.7 mmol/mL/180 min in control patients. Additionally, HbA 1c and fasting glycemia decreased in the treated groups and increased in the control subjects. Daily insulin requirements in the treated groups also decreased compared to those of the control groups. During the follow-up period, severe hypoglycemic events reported by patients were significantly decreased. Limitations of these studies could be a small sample size and the short follow-up period. Moreover, the treated patients did not achieve complete insulin independence. Even so, these results help to improve clinical trial outcomes in future large-scale trials.
Conclusions and perspectives
Stem cell-based therapy has been considered a promising potential therapeutic method for diabetes treatment, especially for T1DM. As mentioned in this review, major advances in research on the derivation of IPCs from hPSCs have improved our chance of reestablishing glucose-responsive insulin secretion in patients with T1DM. However, the clinical trial results of stem cell therapies for T1DM are still dissatisfactory [ 122 ], and many questions and technical hurdles still need to be solved. The major problems include the following four aspects: (1) how to generate more mature functional β-like cells in vitro from hPSCs; (2) how to improve the differentiation efficiency of IPCs from hPSCs; (3) how to protect implanted IPCs from autoimmune attack; (4) how to generate sufficient numbers of desired cell types for clinical transplantation; and (5) how to establish thorough insulin independence. Despite these obstacles, the application of stem cell-based therapy for T1DM represents the most advanced approach for curing type 1 diabetes.
Acknowledgements
Abbreviations, authors’ contributions.
CZ designed the concept. SC wrote the manuscript. SC and KD designed the figures. CZ revised the manuscript. All authors read and approved the final manuscript.
We gratefully acknowledge the funding support from the National Key Research and Development Program of China (2016YFC1305703), the National Natural Science Foundation of China (81670750, 81971191, and 61627807), Guangxi Natural Science Foundation (2014GXNSFDA118030), and the Scientific Research Foundation for the Returned Overseas Chinese Scholars, State Education Ministry.
Availability of data and materials
Ethics approval and consent to participate, consent for publication, competing interests.
The authors declare that they have no competing interests.
Publisher’s Note
Springer Nature remains neutral with regard to jurisdictional claims in published maps and institutional affiliations.
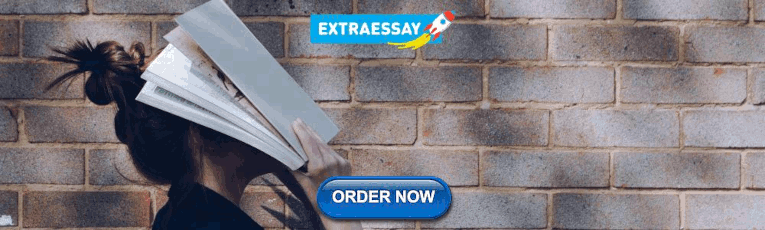
IMAGES
VIDEO
COMMENTS
To accurately identify clinical trials of stem cell transplantation in DM patients, a search was performed using a combination of medical subject heading (MeSH) terms and text words: "diabetes mellitus, type 1" or "diabetes mellitus, type 2" and "stem cell transplantation" and "therapy" or "therapeutic use." Inclusion and Exclusion Criteria
Our hope is that a stem cell-based approach to insulin replacement will ultimately improve glucose control in patients with both type 1 and type 2 diabetes, resulting in healthier, longer lives ...
Towards a stem-cell therapy for diabetes. The isolation of the first human embryonic stem cell (hESC) lines in 1998 opened the possibility of stem cell therapies for a variety of conditions. Type ...
Type 1 diabetes (T1D) is caused by autoimmune destruction of β-cells, whereas type 2 diabetes (T2D) is caused by a hostile metabolic environment that leads to β-cell exhaustion and dysfunction.
Type 2 Diabetes Mellitus (T2DM) accounts for more than 90% of total diabetes mellitus cases all over the world. Obesity and lack of balance between energy intake and energy expenditure are closely linked to T2DM. Initial pharmaceutical treatment and lifestyle interventions can at times lead to remission but usually help alleviate it to a certain extent and the condition remains, thus ...
Journal of Diabetes Investigation is a clinical and experimental diabetes research journal publishing basic science, ... The influence of mesenchymal stem cell therapy on type 2 diabetes in nine trails among 227 patients, including 40 controls, is shown in Figure 1. The articles were reviewed and selected in three stages: reading, screening and ...
Abstract. Since the discovery of insulin a century ago, insulin injection has been a primary treatment for both type 1 (T1D) and type 2 diabetes (T2D). T2D is a complicated disea se that is triggered by the dysfunction of insulin-producing β cells and insulin resistance in peripheral tissues. Insulin injection partially compensates for the ...
Abstract. Objective: To compile and analyze the published studies on cell therapy for type 2 diabetes mellitus (T2DM) to obtain a better insight into management of T2DM that involved stem cell therapy. Methods: We searched all published studies in Pubmed/Medline, and Cochrane library, using keywords: 'stem cell' AND 'therapy' AND ...
Objective: To compile and analyze the published studies on cell therapy for type 2 diabetes mellitus (T2DM) to obtain a better insight into management of T2DM that involved stem cell therapy.
Diabetes mellitus type 2; Insulin; Stem cell treatments; Diabetes; Liver transplantation ... type 2 diabetes is on the rise. A research group has discovered a new gene that may hold the key to ...
Diabetes. 2014. DOI: 10.2337/db13-0717. In this study, scientists NYSCF and Columbia University Medical Center (CUMC) used stem cells created from the skin of patients with a rare form of diabetes—Wolfram syndrome—to elucidate an important biochemical pathway for beta-cell failure in diabetes. NYSCF is turning stem cells from diabetes ...
In type 2 diabetes, beta-cell apoptosis has been suggested to be caused by the formation of toxic oligomers of hIAPP ... Oakley J 2002 Democracy, embryonic stem cell research, and the Roman ...
Harvard Stem Cell Institute (HSCI) scientists are attempting an ambitious, long-term, and high-risk project to create the first animal model for T1D. ... HSCI receives grant from the Crown Prince of Abu Dhabi to help advance type 1 diabetes research. Tuesday, February 8, 2022. Crosstalk between pancreatic cells may drive rare form of diabetes.
In brief, stem cell-derived β-cells or islets hold great potential for diabetes research and treatment, but these findings are based on a limited number of iPSC lines and may not be representative of the entire population, as genetic heterogeneity could influence the results. ... Stem cell-derived islets for type 2 diabetes. Int J Mol Sci ...
Xue et al. engineered and characterized isogenic knockout human embryonic stem cell lines for 20 genes associated with type 2 diabetes risk. Integration of the genomic alterations and subsequent cellular assays in 20 hESC-β cells identifies genes affecting β cell functionality and putative causal variants for type 2 diabetes.
The combined national healthcare costs of Type 1 and Type 2 diabetes exceed $320 billion a year. Current Research Investigators at the Institute for Stem Cell and Regenerative Medicine (ISCRM) are studying the mechanisms that regulate the development and function of beta cells in the pancreas that produce insulin - a key to future treatments ...
1 Department of Endocrinology, Union Hospital, Tongji Medical College, Huazhong University of Science and Technology, Wuhan, China; 2 Hubei Provincial Clinical Research Center for Diabetes and Metabolic Disorders, Wuhan, China; Stem cell-based therapies exhibit considerable promise in the treatment of diabetes and its complications. Extensive research has been dedicated to elucidate the ...
In the long term, the knowledge about C3 can be used to develop new treatments aimed at protecting insulin-producing cells, such as stem cell therapies for treating type 1 diabetes and type 2 ...
Background: Type 2 diabetes mellitus (T2DM), characterized by β-cell dysfunction and insulin resistance (IR), presents considerable treatment challenges. Apelin is an adipocyte-derived factor that shows promise in improving IR; however, it is limited by poor targeting and a short half-life.
Clinical studies on the treatment of type 1 diabetes with device-encapsulated pancreatic precursor cells derived from human embryonic stem cells found that insulin output was insufficient for ...
GLP-1 receptor agonists (GLP-1 RA) are presently used as the first-line drugs for the clinical treatment of type 2 diabetes mellitus (T2DM). It can regulate blood glucose by stimulating insulin secretion and lowering glucagon levels. We used 16S rRNA amplicon sequencing to detect structural changes in the composition of the intestinal flora of newly diagnosed T2DM after 1 and 48 weeks of ...
At a Glance. Researchers found that depleting certain stem cells improved the immune systems of aged mice. The findings suggest that a similar treatment might be used to help protect older people against infections. Hematopoietic stem cells are show dividing in this illustration. The balance of different types of these stem cells, which make ...
MF: Type 1 diabetes was called juvenile diabetes for the longest time, and it was thought to be a disease that had a childhood onset. When diabetes occurred in adulthood it would be type 2 diabetes. But it turns out that approximately half of the cases of Type 1 diabetes may occur during adulthood right past the age of 20 or past the age of 30.
Liam Drew. Encapsulated stem cell-derived islets could shield β cells from the immune system. Credit: Ref. 8. Insulin has been one of the most transformative discoveries in medicine. The ...
With the advent of research on stem cell therapy for various diseases, breakthroughs in stem cell-based therapy for T1DM have been reported. ... type 2 diabetes mellitus (T2DM), gestational diabetes, and monogenic diabetes. Patients with T1DM need daily insulin injections because of the absolute insufficiency of endogenous insulin caused by ...