New Research
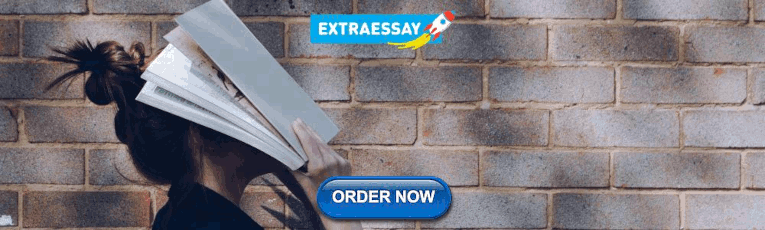
This “Tissue” Paper Is Made From Real Tissue
Made from powdered organs, the flexible paper could be used as a sophisticated bandage during surgery
Jason Daley
Correspondent
/https://tf-cmsv2-smithsonianmag-media.s3.amazonaws.com/filer/8e/ec/8eec902a-29d2-436c-98cf-1487cfb51937/origami_.jpg)
When Adam Jakus was a postdoc at Northwestern University he accidentally spilled some “ink” he'd created from powdered ovaries intended for 3-D printing. Before he could wipe up the mess, it solidified into a thin, paper-like sheet, reports Charles Q. Choi at LiveScience . That led to a lab-bench epiphany.
“When I tried to pick it up, it felt strong,” Jakus says in a press release . “I knew right then I could make large amounts of bioactive materials from other organs. The light bulb went on in my head.”
Jakus, along with the same team that developed a 3-D printed mouse ovary earlier this year, began experimenting with the concept. According to a video , they began collecting pig and cow organs from a local butcher shop, including livers, kidneys, ovaries, uteruses, hearts and muscle tissue.
The team then used a solution to strip the cells from the tissues, leaving behind a the scaffolding material of collagen proteins and carbohydrates. After freeze-drying the matrix, they powdered it and mixed it with materials that allowed them to form it into thin sheets. The research appears in the journal Advanced Functional Materials .
“We’ve created a material we call 'tissue papers' that’s very thin, like phyllo dough, made up of biological tissues and organs,” says Ramille Shah, head of the lab where the research took place, in the video. “We can switch out the tissue we use to make the tissue paper—whether that be derived from liver or muscle or even ovary. We can switch it out very easily and make a paper out of any tissue or organ.”
According to the press release, the material is very paper-like and can be stacked in sheets. Jakus even folded some into origami cranes. But the tissue paper’s most important property is that it is biocompatible and allows for cellular growth. For instance, the team seeded the paper with stem cells, which attached to the matrix and grew over four weeks.
That means the material could potentially be useful in surgery, since paper made of muscle tissue could be used as a sophisticated Band-Aid to repair injured organs. “They're easy to store, fold, roll, suture and cut, like paper," Jakus tells Choi. “Their flat, flexible nature is important if doctors want to shape and manipulate them in surgical situations.”
Northwestern reproductive scientist Teresa Woodruff was also able to grow ovary tissue from cows on the paper, which eventually began producing hormones. In the press release, she explains that a strip of the hormone-producing tissue paper could be implanted, possibly under the arm, of girls who have lost their ovaries due to cancer treatments to help them reach puberty.
The idea of using extracellular matrices, hydrogels or other material as a scaffolding to bioprint organs like hearts and kidneys is being investigated by labs around the world. In 2015, a Russian team claimed they printed a functional mouse thyroid . And this past April, researchers were able to b ioprint a patch derived from human heart tissue that they used to repair the heart of a mouse.
Get the latest stories in your inbox every weekday.
Jason Daley | | READ MORE
Jason Daley is a Madison, Wisconsin-based writer specializing in natural history, science, travel, and the environment. His work has appeared in Discover , Popular Science , Outside , Men’s Journal , and other magazines.
Increasing the availability of quality human tissue for research
Affiliations.
- 1 Physicians Committee for Responsible Medicine, Washington, DC, USA.
- 2 LifeNet Health, Virginia Beach, NC, USA.
- 3 Amnion Foundation, Winston-Salem, USA.
- 4 International Institute for the Advancement of Medicine, Edison, NJ, USA.
- 5 University of Minnesota, Minnesota, MN, USA.
- PMID: 33080036
- DOI: 10.14573/altex.2007141
Advances in 3D and other in vitro tissue model platforms have led to fundamental improvements in research on human disease, development of novel therapies, and safety testing. In addition, histological and cellular investigations of human tissues continue to serve as keystones in understanding disease and health processes. In recognition of the importance of human tissues in research, the Physicians Committee for Responsible Medicine held a workshop. Working closely with key stakeholders from the research community, regulatory agencies, and organ procurement organizations, the goal was to explore, understand, and address the barriers to increased use of human organs, tissues, and cells in research. Workshop participants were tasked with identifying the challenges of accessing and qualifying tissues for research purposes and creating a strategy to help meet the needs of the research communities to increase the availability and quality of human tissues in biomedical and translational research. Break-out groups identified significant challenges in the areas of policy, scientific development, and public engagement with respect to the provision and application of tissues and cells for scientific advancement. Following working group recommendations, stakeholders concluded that there is a need to facilitate the availability and quality of human tissues for the research community, as well as provide a framework for education of the public, medical professionals, and researchers to foster donation and utilization for research in place of animal models. The success of these new initiatives will facilitate greater access to high-quality human tissues for biomedical and translational research and help ensure the transition away from the dependence on animal models.
Publication types
- Biomedical Research
- Tissue Culture Techniques / methods*
- Tissue Culture Techniques / standards*
- Search Menu
- Advance Articles
- Clinical Case Studies
- Journal Club
- Clinical Chemistry Podcasts
- Clinical Trainee Council
- Special Issues
- Clinical Chemistry Guide to Scientific Writing
- Clinical Chemistry Guide to Manuscript Review
- Author Guidelines
- Submission Site
- Self-Archiving Policy
- Call for Papers
- Why Publish?
- About Clinical Chemistry
- Editorial Board
- Advertising & Corporate Services
- Journals on Oxford Academic
- Books on Oxford Academic
Article Contents
Governing treaties, laws, and regulations, informed consent, specimens obtained postmortem, future considerations, conclusions, 4 nonstandard abbreviations:, acknowledgments.
- < Previous
Human Tissue Ownership and Use in Research: What Laboratorians and Researchers Should Know
- Article contents
- Figures & tables
- Supplementary Data
Monica J Allen, Michelle LE Powers, K Scott Gronowski, Ann M Gronowski, Human Tissue Ownership and Use in Research: What Laboratorians and Researchers Should Know, Clinical Chemistry , Volume 56, Issue 11, 1 November 2010, Pages 1675–1682, https://doi.org/10.1373/clinchem.2010.150672
- Permissions Icon Permissions
The use of human blood and tissue is critical to biomedical research. A number of treaties, laws, and regulations help to guide the ethical collection of these specimens. However, there are no clearly defined regulations regarding the ownership of human tissue specimens and who can control their fate.
This review discusses the existing regulations governing human studies and the necessary components of patient consent. Legal cases that have addressed the issue of ownership of human tissue are reviewed, including recent settlements that have led to the destruction of millions of specimens of patient tissue. The unique regulations that guide the use of tissues collected postmortem are also examined. Potential changes in the future of biomedical research that uses human tissue, including genetic material, are also discussed.
The use of human tissue is directed by numerous laws and regulations. Awareness of these rules and of how and when to obtain meaningful informed consent from patients is essential for laboratorians and researchers, who should also be familiar with situations that have led to lawsuits and in some cases the destruction of valuable human tissue specimens.
The study of the human body and its tissues dates back to ancient Greece. Unfortunately, after the fall of the Roman Empire, anatomical studies came to a near standstill and in many places the use of cadavers became illegal. For many years researchers were prosecuted for postmortem dissections. It wasn't until the 15th century that researchers at medical schools in Europe were able to study the human body and its tissues without the fear of prosecution ( 1 ). Human studies have come a long way since then, and tissue samples have become critical to the research enterprise.
Research specimens are obtained from the following four sources: ( a ) tissues collected prospectively for a research project; ( b ) excess tissue from samples taken specifically for clinical purposes, such as diagnosis or treatment, which are subsequently recognized as valuable for research; ( c ) cadaveric tissues; and ( d ) tissues with reproductive or “human” potential, including eggs, sperm, zygotes, embryos, and fetal tissues, which are also often collected for clinical purposes, as in ( a ). With the increased use of human tissue in medical research, researchers, research institutions, and human research participants have asked: Who gets to determine the fate of such specimens? In the US, a country that prides itself on property rights, this question has prompted another: Who “owns” human tissue specimens? This question has been at the heart of several closely watched court cases.
In this review we explore the governing treaties, laws, and regulations that guide human studies; the necessary components of informed consent; legal cases that have examined the issue of ownership of human specimens; and the unique situation of specimens obtained postmortem. We also provide a brief look into the future of research that uses human tissue.
To understand the court rulings in legal cases that have involved the use of human specimens, it is important to be familiar with treaties, laws, and regulations that govern human research. Most aspects of the interactions between research and human research participants are heavily controlled by federal regulation, although it is important to note that these regulations do not address the issue of ownership. The laws governing the use of human research participants have their origin in the Declaration of Helsinki, which was developed by the World Medical Association as a set of ethical principles regarding human experimentation ( 2 ). The Declaration of Helsinki was the first important effort of the medical community to regulate such research. The Declaration of Helsinki is not a legally binding instrument in international law, but it has greatly influenced national legislation and regulations. The Declaration of Helsinki was originally adopted in 1964 and has since undergone 6 revisions. In the US, the principles set forth in the Declaration of Helsinki are embodied in the Common Rule.
The Common Rule is a set of regulations within the Code of Federal Regulations (CFR). 4 The CFR is a set of rules and regulations established by the US government to add regulatory guidance to the congressionally enacted statutes found in the United States Code. The regulation that addresses the protection of human research participants is referred to as the Common Rule. Sixteen federal agencies have adopted the Common Rule in the form set forth by the Department of Health and Human Services (HHS) in Title 45 (public welfare) part 46 (protection of human subjects) ( 3 ). The federal Food and Drug Administration (FDA) has adopted a slightly different version of the Common Rule (21 CFR part 50) that allows the FDA to concurrently enforce regulations that protect human study participants in the conduct of studies generating drug, device, or in vitro diagnostic data that will be submitted to the FDA ( 4 ).
The Common Rule is coordinated, interpreted, and enforced largely by the Office of Human Research Protection, which is a division of the HHS. Institutions engaged in research with human participants that is conducted or supported by HHS must submit a Federalwise Assurance to the Office of Human Research Protection stating that the institution will comply with the human research participant protection regulations of all federal agencies. Most universities have agreed to apply the Common Rule to all human research, not just studies supported by federal dollars.
The Common Rule sets forth, in detail, the composition, function, and role of institutional review boards (IRBs) in protecting human participants in research activities. The Common Rule also outlines the requirements for obtaining informed consent from human research participants. In addition, HHS has adopted additional protections for special research groups such as pregnant patients, fetuses, neonates, children, and prisoners. The Common Rule does not address the question of who owns human tissue specimens used in research. It also does not apply to tissue obtained postmortem, though the Health Insurance Portability and Accountability Act of 1996 (HIPAA) does regulate the use of information associated with those tissues.
Some states have also enacted laws governing research with human participants. A comprehensive review of state laws is beyond the scope of this article. However, it is important to know that state law may provide additional protections, and should be consulted.
What are the required elements of informed consent? First, the researcher must provide the individuals who participate in research studies with an explanation of the purposes of the research and the expected duration of the individual's participation. General descriptions are not sufficient; descriptions must be specific to the study ( 3 , 5 ). Participants in research cannot give “informed” consent if they are not adequately informed about the intended purpose of the research. Transparency is a key element in the consent process. Study participants must be informed of all intended uses for their specimens. If the use of specimens in additional research is desired later, study participants must give additional informed consent for this new research, or specimens must be deidentified (see below). Secondary research on specimens is also permitted if the IRB waives informed consent for the secondary project. IRB waiver is more likely if, at the time of tissue collection, the study participant consented to future research.
The consent information provided to study participants must contain an adequate description of the risks and potential benefits to the participants or others, any alternatives to participation in the study, and what the participant is expected to do throughout the study (including any costs associated with the study). A statement is required that describes the extent, if any, to which confidentiality of records that identify the study participant will be maintained. For research that involves more than minimal risk, the study participant must be informed whether any compensation and medical treatments are available if injury occurs. It must be clear that participation is voluntary and that the study participant may withdraw at any time without penalty. Finally, the informed consent document must provide information on a person who can be contacted if the research participant has questions or suffers a research-related injury ( 3 ).
The Common Rule does permit research without the consent of the research participant in certain circumstances. First, the Common Rule applies only to human research participants, termed “human subjects,” defined as living individuals with whom the investigator interacts or about whom the investigator obtains identifiable private information ( 3 ). Therefore, if research is conducted by using anonymized or deidentified samples only and the researchers do not have access to patients' private information, then this research, by definition, would not be human subject research and would not require informed consent.
In addition, the Common Rule (and its informed consent requirement) does not apply to research conducted on existing pathological or diagnostic specimens, if the IRB determines that the research is exempt because the information will be recorded by the researcher in a way that does not permit identification of the research participant ( 3 , 6 ).
Finally, the IRB has discretion to waive or alter the informed consent requirements if the IRB finds and documents that: ( a ) the research involves no more than minimal risk to the study participants, ( b ) the waiver or alteration will not adversely affect the rights and welfare of the study participants, ( c ) the research could not practicably be carried out without the waiver or alteration, and ( d ) whenever appropriate, the study participants will be provided with additional pertinent information after participation ( 3 ).
Although the use of human tissue is heavily regulated by the federal government, the question of who owns excised human tissue has been analyzed under state property law. In a number of cases, courts have considered the question of whether an individual retains an ownership interest in his/her excised tissue that would authorize that person to share in the profits of any commercialization of research results, dictate who controls the samples, or determine how and if the sample will be used in future research.
In some cases, the debate has been framed as one of tissue “guardianship” (or bailment) vs “ownership” ( 7 ). Bailment describes a legal relationship in which physical possession of personal property is transferred from one person (the “bailor”) to another person (the “bailee”), who subsequently holds the property for the benefit of the bailor and is subject to the bailor's right to reclaim possession at any time. Bailment is distinguished from a sale or a gift of property, because bailment involves only the transfer of possession, not ownership. Property owners generally have the right to use, sell, transfer, exchange, or destroy their property as they wish, and to exclude others from doing these things; bailees do not have similar rights in bailed property. In the context of research specimens, the question is whether the transfer of excised tissue to a research institution is a gift, a bailment, or something in between.
In most cases involving tissue excised for clinical purposes and tissue donated for research, courts have concluded that patients and other research study participants do not retain ownership rights of the excised tissue. Contrary rulings have been reached in cases in which the evidence showed that there was a clear understanding that the patient would retain ownership of the excised tissue. Table 1 contains a list of important cases that have dealt with specimen ownership.
Important cases regarding specimen ownership.
The seminal case on this question is Moore v. Regents of University of California , which was decided by the Supreme Court of California in 1990. In 1976, Moore underwent a splenectomy at the University of California to treat his hairy cell leukemia. Between 1976 and 1983, Moore traveled to the University of California from his home in Seattle several times. He claimed that he did so believing that he required ongoing treatment. He later learned that the university was conducting research on material obtained during his treatment and had created a cell-line using that material ( 7 , 8 , 9 ). The cell line was subsequently patented and used by the University of California for commercial gain.
Moore asserted a variety of claims, including conversion (when a party takes away or wrongfully assumes the right to goods which belong to another) and lack of informed consent. In his conversion claim, Moore contended that he had an ownership interest in his cells, and the University of California took them unlawfully. The court dismissed the conversion claim, holding that current state law did not support a conversion claim and creating such a claim would unreasonably burden medical research ( 7 , 8 ). The court did, however, find that Moore could proceed on his claim that the doctors had breached their fiduciary duty to obtain informed consent because they failed to disclose their research interest and the economic benefit associated with the additional (and perhaps unnecessary) procedures they performed on him. The court drew a distinction between the privacy and dignity interests protected by the informed consent doctrines, and property rights.
These issues were addressed a decade later in Greenberg v. Miami Children's Research Hospital Institute . In 1987, the father of 2 children with Canavan disease worked with a researcher named Reuben Matalon to set up a registry of affected families to collect tissue from willing donors to begin studying the molecular basis of the disease. With the families' support, Matalon found a Canavan gene and developed a genetic test. In 1997, Matalon's employer, Miami Children's Hospital, obtained a patent on the gene and began licensing a test to identify Canavan mutations. Four families and 3 nonprofit organizations filed suit, alleging that Matalon and Miami Children's Hospital used the children's tissue without consent to license a patent and develop a commercial test ( 10 ). They claimed, among other things, that they had an ownership interest in the excised tissue and that the defendants “converted” the tissue for their own economic benefit. The court found that the tissue was given voluntarily for research without any expectation of return, and therefore the plaintiffs had no ownership interest in the tissues, or the research performed using the tissue ( 7 , 10 ). The court noted that a contrary rule would cripple medical research because it would “bestow a continuing right for donors to possess the results of any research conducted by the hospital.”
More recently, William Catalona, a urologic surgeon from Washington University School of Medicine, sought a court order directing the university to send the contents of a tissue repository to him at his new employer. The repository contained more than 100 000 serum samples, 3500 prostate tissue samples and 4400 DNA samples that had been collected (via an informed consent process) over a 20-year period from volunteers, including patients of Catalona and his colleagues in the urology division ( 9 , 11 ). The donated material was made available for Catalona and other colleagues for the purpose of conducting research on prostate cancer. When Catalona decided to leave Washington University in 2003, he wrote a letter to the sample donors asking that they sign a form to “release” the samples to him. Approximately 6000 study participants signed the form. However, Washington University refused to transfer the samples, arguing that they were the property of the university. The US District Court for the Eastern District of Missouri considered the informed consent documents signed by the donors at the time of tissue donation, the testimony of witnesses, and relevant federal guidelines, and concluded that the samples legally remained the property of Washington University. The US Court of Appeals for the Eighth Circuit affirmed the decision, holding that whatever interest the sample donors might continue to have by virtue of the specific language in the consent documents (such as a right to request that the samples be destroyed) or by virtue of the Common Rule (such as the right to withdraw participation from research), they could not ask that the samples be transferred to a different facility ( 7 , 8 ). The court noted that the samples could not legally be returned to the donors under laws governing the proper handling and disposal of biological waste—a fact that significantly undermined the donors' claim of ownership.
Taken together, these court rulings suggest that patients and other human research participants do not retain ownership interests in their excised tissue. The tissue donors cannot benefit economically from research performed on that tissue and they cannot require the receiving institution to transfer the tissue to a site of their choosing. Courts have been reluctant to burden medical research in these ways. At the same time, it is clear that the tissue donor does retain certain rights in the tissue. For example, depending on how the informed consent documents are structured, some donor “property”-like rights may be reserved, such as the ability to direct destruction of the donated tissue after the donation is made. And nothing in these cases obviates the researcher's obligation to obtain informed consent from the tissue donor, in cases in which informed consent is required, before the use of information that personally identifies the donor in subsequent research on donated samples.
The outcomes of these cases also demonstrate that the ownership question does not depend on whether a patient consented to the use of his/her excised tissue for research. Although the Moore court did not condone the physician's failure to obtain informed consent, and permitted Moore to assert a claim against his physician based on that failure, these facts did not alter the court's determination that Moore had no ownership interest in the excised tissue. This approach is consistent with the commonplace practice of using leftover material obtained during routine medical or diagnostic procedures for future research purposes. Such material is usually stored according to guidelines from the College of American Pathologists and the Joint Commission. These guidelines include distinctions between leftover serum samples, which are usually disposed of in a short, predetermined amount of time after collection, and paraffin-blocked tissues, which are often retained for years and are considered by some to be part of the patient's medical record. For instance, according to the College of American Pathologists, leftover urine should be stored for 24 h; serum, plasma, and cerebrospinal and other body fluids for 48 h; and peripheral blood smears for 7 days, whereas paraffin blocks, wet tissue, and fine-needle aspiration specimens on slides are to be stored for 10 years. This stored material is often used in medical research. State laws also exist that dictate how long diagnostic material must be stored by the laboratory as “guardian” ( 12 ).
Currently, no laws or regulations exist regarding ownership of these leftover materials. Many bioethicists consider leftover diagnostic tissues to be “abandoned” by patients, and conclude that the patient has relinquished any property rights over the material. The basis of this concept is that the donor has no further property interest in the leftover material, particularly if it is diseased or no longer necessary for human functions ( 6 ). This argument is especially applicable to excess blood specimens or tissues that would normally be discarded if they were not put to an alternate use. Even if the donor has no continuing property right, the laboratory must abide by ethical and legal guidelines if this material is to be used for research.
The Common Rule authorizes the use of such materials if the information is recorded in a manner that it does not permit identification of the individual from whom the material was obtained, either directly or through the use of identifiers that are linked to the patient ( 3 ). Utilization of these leftover materials for research requires IRB approval, and the IRB has authority to waive patient consent when appropriate. In addition, federal law and HIPAA guidelines must be followed to ensure that these materials are deidentified and/or the patients' protected health information remains secure.
A different analysis has been applied when the patient has a continuing use for the excised tissue. In York v. Jones (1989), a couple entering into an in vitro fertilization (IVF) program signed a cryopreservation agreement for the freezing of their fertilized eggs ( 7 ). Later, the couple sought treatment at another hospital and asked that their prezygotes be transferred to that facility. The defendants argued that under the agreement, the Yorks' property rights were limited to implantation, donation to another infertile couple, donation for approved research, or thawing, and that interinstitutional transfer was not an option. The court disagreed, noting that the agreement consistently referred to the prezygotes as the Yorks' “property” and that the contractual limitations on the Yorks' rights were only applicable if they no longer desired to initiate a pregnancy ( 7 ). The property issues in this case are distinctive because, unlike leftover blood or tissue, the primary intent of an IVF program is to return the prezygote to the couple via an IVF procedure. It is also clear that a variety of factors influence the determination of legal ownership of bodily tissues, including the particular terms of informed consent documents and other agreements.
It is increasingly clear that although donors of research specimens do have continuing rights regarding the use and secondary use of their samples, they do not own those samples or control their disposition. Interestingly, however, 2 recent court cases arising from disputes about researchers' use of samples have led to the destruction of patient specimens. The first case, Baleno et al. v. Texas Department of State Health Services , involved more than 5 million leftover dried blood-spot samples collected for newborn screening by the Texas Department of State Health Services (DSHS). According to a lawsuit filed by 5 plaintiffs, the state had been retaining these samples since 2002 for use in research. The plaintiffs claimed that defendants had violated plaintiffs' rights under the Fourth Amendment to the US Constitution to be free of unreasonable searches and seizures, because consent was not obtained for indefinite storage and undisclosed research, and the defendants had effectively made the samples their own property. Plaintiffs also claimed that the blood spots contained deeply private medical and genetic information, and defendants' retention and use of the samples violated plaintiffs' rights to privacy and liberty under the 14th Amendment. In response to the lawsuit, the Texas legislature enacted a law governing the collection of newborn blood samples. The law states that the Texas DSHS may retain the leftover material for research as long as parents are given an opportunity to “opt out” by filling out a “destruction directive.” Shortly thereafter, the lawsuit was settled, and DSHS agreed to destroy the remaining specimens in their bank, although the 10–12 000 blood spots already released to some 35 research projects could continue to be used ( 13 ). As a result of this case the American College of Medical Genetics released a “Position Statement on Importance of Residual Newborn Screening Dried Blood Spots.” In this statement the college underscores the value of these specimens and urges states to save them with the utmost respect for privacy and confidentiality ( 14 ).
In the second recent case, members of the Havasupai tribe in Arizona sued Arizona State University. The plaintiffs alleged that, in 1990, they had consented to use of their blood samples for diabetes research. However, their DNA was also being used for studies on schizophrenia, metabolic disorders, alcoholism, inbreeding, and population migration ( 15 ). Plaintiffs alleged a host of claims, including breach of fiduciary duty, lack of informed consent, and conversion. Recently, the Arizona State University Board of Regents agreed to pay $700 000 to the tribe members, provide other forms of assistance to the impoverished Havasupai tribe, and return the blood samples ( 16 ).
In both of these cases, the plaintiffs' chief complaint was that the researchers were not transparent about what they intended to do with these patient specimens and did not obtain proper consent. Because the cases were settled privately before court rulings, we do not know how a court would have ruled on the plaintiffs' allegations. It is possible, however, that with greater transparency these lawsuits would have been avoided.
A different question can arise when researchers use tissue obtained postmortem. That is, the question of whether family members have an ownership interest in the decedent's body. The Common Rule does not apply to tissues donated postmortem because that regulation applies only to the research participation of living individuals. The Uniform Anatomical Gift Act (UAGA) gives individuals the right to execute documents that provide for donation of their own organs for transplantation and or their bodies for use in the study of medicine ( 17 ). The UAGA also provides that in the absence of such a document, a surviving spouse, or if there is no spouse, a hierarchical list of specific persons, can make the gift. The law also seeks to limit the liability of healthcare providers who act on good faith representations that a deceased patient indicated the intention to make an anatomical gift. All states have adopted some version of the UAGA. Nearly 40 states have enacted the most recent (2006) revisions or have legislation pending to do so.
Like the Common Rule, the UAGA does not address the question of ownership, and different courts have reached different conclusions on the question of whether a family member has an ownership interest in a relative's cadaver. For example, in Mansaw v. Midwest Organ Bank , a father claimed that his property rights were violated when a hospital harvested his son's organs without his consent ( 18 ). The UAGA required only the consent of 1 parent, and the mother had agreed to the donation. The court concluded that the father and mother were coowners of their son's body. However, that property right was minimal, and the UAGA provision permitting a single parent (and just 1 of the coowners) to dispose of the “property” did not violate the father's rights. Alternatively, in Adams v. King County , under a different set of circumstances, the court came to a different conclusion on the property issue ( 19 ). In that case, the family of a 21-year old organ donor, Jesse Smith, sued the King County Coroner's office when the family learned that instead of being made available for transplantation, their son's brain, liver, and spleen had been removed and sent to the Stanley Medical Research Institute in Baltimore, Maryland. Smith's brain was used in a schizophrenia study. According to Smith's family, Smith had never expected his donated organs to be used for anything other than organ transplantation. The court permitted the family to assert a claim against the research institute based on the family's interest in proper treatment of the body and the mental suffering caused by misuse of the body. However, the court disagreed with Mansaw v. Midwest Organ Bank , and specifically stated that the family's interest was not a property interest.
The law regarding donor control over excised tissue samples is still evolving. The cases have generally rejected claims that patients and other human research participants retain property interests in excised tissue. There are ongoing efforts, however, to address questions about the donors' right to control the future use of that tissue. There is much discussion regarding how to obtain informed consent. Several different approaches have been proposed, including specific consent, tiered consent, general permission, and presumed consent ( 20 ). Each approach has advantages and disadvantages. Researchers will need to decide which type of consent is best for their needs. The reader is directed to a recent discussion of these approaches by Mello and Wolf ( 20 ).
Mello and Wolf suggest that 2 key questions remain: First, could a 1-time general (also called blanket or global) consent to all future research be used? It is not clear, however, if this type of consent would comport with HIPAA because HIPAA requires project-specific consent for use of the patient's protected health information in research, unless the IRB has made a specific determination to the contrary. Second, does removal of an individual's indentifying information from tissue samples really alleviate the risk and ethical obligations to that individual ( 20 )? Just because samples are deidentified does not necessarily mean that the donor does not object to the proposed research.
State legislatures may also address these issues. California has new funding regulations that require researchers to honor the donor's requests regarding the types of regenerative manipulations that can be done to tissue—even if the tissue has been anonymized ( 8 ). These regulations impose obligations greater than those imposed by the Common Rule, which does not require informed consent for research on anonymized samples.
Charo has argued that if one's body is property then uninvited removal of specimens or even the uninvited use of specimens could constitute theft or trespassing ( 8 ). This uninvited removal or use could also be considered an injury and a “deprivation of liberty.” In an interesting perspective piece, Hakimian and Korn ( 18 ) pointed out that if specimens are treated as property many new questions are raised. Is a person entitled to sell their specimens and organs? Do their specimens and organs become the property of their heirs and could they profit from their sale? Or, should bodies and tissues be viewed as part of a “common heritage of humanity, to be used for the collective good”? This approach would suggest that (assuming patient privacy is protected and their liberties are not deprived) that the public has a right to excised specimens. In fact, the American Medical Association and the HHS Advisory Committee on Organ Transplantation have considered a “presumed consent” system for organ donation ( 19 ). This would assume that everyone can be considered an organ donor unless they “opt out” and explicitly express their option not to donate. A number of European countries already operate on this opt out system. These types of programs certainly operate on the premise of collective good and public rights. Other authors ( 21 ) have argued that biospecimen banks should be set up as charitable trust agreements, in which the donors transfers their property rights to the trust. In this model the general public acts as the beneficiary and hospitals act as stewards rather than brokers. Perhaps models such as these will minimize the legal battles over human tissue use in biomedical research.
The likelihood that a patient will make claims against researchers who are using tissues that would normally be discarded is probably low. However, as studies on genetic material become more prevalent, the frequency of lawsuits may increase. A list of what laboratorians and researchers should do before conducting research on human tissue specimens is given in Table 2 . Researchers should strive for transparency in what they intend to do with donated human tissue and should protect the privacy of tissue donors. Informed consent should be obtained from individuals who provide tissue specimens whenever required. If new studies are undertaken on specimens, IRB approval should be obtained for each new study to ensure that the new research is covered by the intent of the original signed consent. Researchers should avoid using specimens for research that was not outlined in the consent form. In cases for which consent is not required, maintaining the privacy and confidentiality of the tissue donors is of the utmost importance. Finally, as the guardians of tissue obtained both prospectively for tissue repositories and “abandoned” samples obtained during treatment, clinical laboratorians and surgical pathologists should be cognizant of any local laws governing use of that tissue.
What laboratorians and researchers should do before conducting research on human tissue specimens.
Code of Federal Regulations
Department of Health and Human Services
institutional review board
Food and Drug Administration
Health Insurance Portability and Accountability Act of 1996
in vitro fertilization
Department of State Health Services
Uniform Anatomical Gift Act.
Author Contributions: All authors confirmed they have contributed to the intellectual content of this paper and have met the following 3 requirements: (a) significant contributions to the conception and design, acquisition of data, or analysis and interpretation of data; (b) drafting or revising the article for intellectual content; and (c) final approval of the published article.
Authors' Disclosures of Potential Conflicts of Interest: Upon manuscript submission, all authors completed the Disclosures of Potential Conflict of Interest form. Potential conflicts of interest:
Employment or Leadership: None declared.
Consultant or Advisory Role: None declared.
Stock Ownership: None delcared.
Honoraria: None declared.
Research Funding: None declared.
Expert Testimony: None declared.
Other: M.J. Allen, counsel of record in the Catalona case.
Role of Sponsor: The funding organizations played no role in the design of study, choice of enrolled patients, review and interpretation of data, or preparation or approval of manuscript.
We thank Nancy Pliski for her help in preparing this manuscript.
Malomo AO , Idowu OE , Osuagwu FC . Lessons from history: human anatomy, from the origin to the renaissance . Int J Morphol 2006 ; 24 : 99 – 104 .
Google Scholar
World Medical Association (WMA) . WMA Declaration of Helsinki: ethical principles for medical research involving human subjects . http://www.wma.net/en/30publications/10policies/b3/index.html (Accessed August 2010).
US HHS . Code of Federal Regulations. Title 45: Public Welfare. Part 46: Protection of human subjects . http://www.hhs.gov/ohrp/humansubjects/guidance/45cfr46.htm (Accessed August 2010).
US HHS . Code of Federal Regulations. Title 21 . http://www.accessdata.fda.gov/scripts/cdrh/cfdocs/cfcfr/CFRsearch.cfm?CFRPart=50 (Accessed August 2010).
Wendler D . One-time general consent for research on biological samples . Arch Int Med 2006 ; 166 : 1449 – 52 .
Lazzarini Z , Case P , Thomas CJ . A walk in the park: a case study in research ethics . J Law Med Ethics 2009 : 93 – 103 .
Andrews L . Who owns your body? A patient's perspective on Washington University v. Catalona . J Law Med Ethics 2006 : 398 – 407 .
Charo RA . Body of research: ownership and use of human tissue . N Engl J Med 2006 ; 355 : 1517 – 9 .
Glantz L , Roche P , Annas GJ . Rules for donations to tissue banks—what next? N Engl J Med 2008 ; 358 : 298 – 303 .
Marshall E . Genetic testing: families sue hospital, scientist for control of Canavan gene . Science 2000 ; 290 : 1062 .
Kaiser J . Biomedical research: court decides tissue samples belong to university, not patients . Science 2006 ; 312 : 346 .
Dry S . Who owns diagnostic tissue blocks? Lab Med 2009 ; 40 : 69 – 73 .
Doerr A . Newborn blood spot litigation: 70 days to destroy 5+ million samples . Genomics Law Report 2010 . http://www.genomicslawreport.com/index.php/2010/02/02/newborn-blood-spot-litigation-70-days-to-destroy-5-million-samples/ (Accessed August 2010).
American College of Medical Genetics . Position statement on importance of residual newborn screening dried blood spots . http://www.acmg.net/StaticContent/NewsReleases/Blood_Spot_Position_Statement2009.pdf (Accessed August 2010).
Andrews L . The Havasupai case: research without patient consent . http://www.whoownsyourbody.org/havasupai.html (Accessed August 2010).
Harmon A . Indian tribe wins fight to limit research of its DNA . New York Times 2010 Apr 21 . http://www.nytimes.com/2010/04/22/us/22dna.html (Accessed September 2010).
The National Conference of Commissioners of Uniform State Laws . Uniform anatomical gift act . http://www.anatomicalgiftact.org/DesktopDefault.aspx?tabindex=1&tabid=63 (Accessed August 2010).
Hakimian R , Korn D . Ownership and use of tissue specimens for research . JAMA 2004 ; 292 : 2500 – 5 .
Cooper S . Informed consent and malformed consent . American Thinker . http://www.americanthinker.com/2007/06/informed_consent_and_malformed.html (Accessed August 2010).
Mello MM , Wolf LE . The Havasupai Indian tribe case: lessons for research involving stored biologic samples . N Engl J Med 2010 ; 363 : 204 – 7 .
Winickoff DE , Winikoff RN . The charitable trust as a model for genomic biobanks . N Engl J Med 2003 ; 349 : 1180 – 4 .
Supplementary data
Email alerts, citing articles via.
- Recommend to Your Librarian
- Advertising and Corporate Services
- Journals Career Network
Affiliations
- Online ISSN 1530-8561
- Print ISSN 0009-9147
- Copyright © 2024 Association for Diagnostics & Laboratory Medicine
- About Oxford Academic
- Publish journals with us
- University press partners
- What we publish
- New features
- Open access
- Institutional account management
- Rights and permissions
- Get help with access
- Accessibility
- Advertising
- Media enquiries
- Oxford University Press
- Oxford Languages
- University of Oxford
Oxford University Press is a department of the University of Oxford. It furthers the University's objective of excellence in research, scholarship, and education by publishing worldwide
- Copyright © 2024 Oxford University Press
- Cookie settings
- Cookie policy
- Privacy policy
- Legal notice
This Feature Is Available To Subscribers Only
Sign In or Create an Account
This PDF is available to Subscribers Only
For full access to this pdf, sign in to an existing account, or purchase an annual subscription.
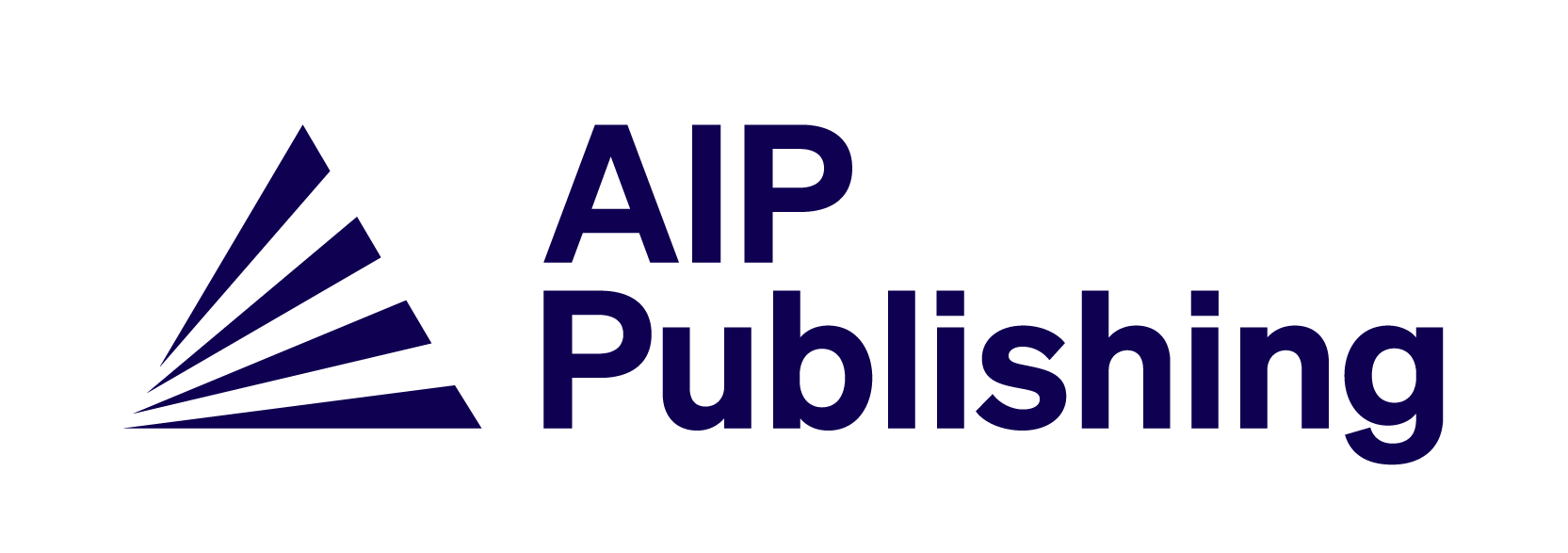
- Previous Article
- Next Article
Utilization of corn husk for tissue papermaking
- Article contents
- Figures & tables
- Supplementary Data
- Peer Review
- Reprints and Permissions
- Cite Icon Cite
- Search Site
Natalia Suseno , Marisca E. Gondokesumo , Puspita R. Permatasari; Utilization of corn husk for tissue papermaking. AIP Conf. Proc. 11 November 2021; 2338 (1): 040019. https://doi.org/10.1063/5.0067417
Download citation file:
- Ris (Zotero)
- Reference Manager
The demand of tissue papers is increasing with the population increase. This will definitely increase the need of wood fibers as the main raw material. However, due to the wood shortages, there have been many attempts to use non- wood fibers as substitutes for papermaking. In Indonesia, corn production has gradually increased for the last 5 years, hence it also has an impact on the raising in the amount of corn husk waste. Corn husk has a high cellulose content which suitable to be used as a raw material for tissue papermaking. In this experiment, soda pulping process was conducted to remove out lignin. The resulting tissue paper will be added with additives that have antimicrobial properties of chitosan and mangosteen peel for the purpose of increasing the tensile strength or absorption of water. The aim of this research is to study the effect of depending variables (temperature and NaOH concentration) on chemical composition (cellulose and lignin content), and physical properties including water absorption and tensile strength.The research was started with the initial process of removing the lignin content in the pulp by pretreating delignification using the sodium hydroxide (NaOH) process with several variations in concentration (4–10%), and temperature (60–90°C) for 1.5 hours. To obtain tissue with a good physical condition, it has been influenced by the optimum chemical composition containing high cellulose and low lignin content, high tensile strength and water absorption. The optimum conditions for tissue paper in this study were at 90°C and 4% of NaOH concentration. The next step will be to vary the composition of the additive in order to obtain the effect of physical properties (tensile strength and water absorption).
Sign in via your Institution
Citing articles via, publish with us - request a quote.
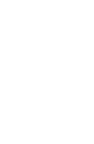
Sign up for alerts
- Online ISSN 1551-7616
- Print ISSN 0094-243X
- For Researchers
- For Librarians
- For Advertisers
- Our Publishing Partners
- Physics Today
- Conference Proceedings
- Special Topics
pubs.aip.org
- Privacy Policy
- Terms of Use
Connect with AIP Publishing
This feature is available to subscribers only.
Sign In or Create an Account

Please fill the all required fields....!!
World of TISSUES

The tissues sector has boomed over the last few years. With a move to more luxurious tissue paper and ultra absorbent paper towels the industry has been able to increase the tissue prices and create new brands to retain consumers. Add to this growing market in developing countries and a drive to innovate, and there are very positive signals for the future of tissues. The tissue segment has become one of the most important segments of the paper industry in developed countries. It produces one of the most highly valued and appreciated, though not necessarily talked about, paper products.
Tissue paper or simply tissue is a lightweight paper or, light crêpe paper. Tissue can be made both from virgin and recycled paper pulp. Tissue papers are the most common thing that we use in our daily life from cleaning, dusting, wrapping and personal use.
As far as the history of tissue paper industry goes, although paper had been known as a wrapping and padding material in China since the 2nd century BC, the first use of toilet paper in human history dates back to the 6th century AD, in early medieval China. If we could travel back in time to 1391, we would encounter a Chinese emperor who demanded the first paper sheets sliced to be placed in his outhouse. The first “official” toilet paper was introduced in China measuring a whopping 2 ft X 3 ft each.
An important move towards the production and distribution of modern toilet tissue paper came from a teacher in Philadelphia in 1907. Concerned about a mild cold epidemic in her classroom, she blamed it on the fact that all students used the same cloth towel. She proceeded to cut up paper into squares to be used by her class as individual towels, a revolutionary idea. Arthur Scott of Scott Paper Company heard about this teacher and decided he would try to sell the carload of paper. He perforated the thick paper into small towel-size sheets and sold them as disposable paper towels. Later he renamed the product Sani-Towel and sold them to hotels, restaurants, and railroad stations for use in public washrooms.
In 1931, Scott introduced the first paper towel for the kitchen and created a whole new grocery category. He made perforated rolls of "towels" thirteen inches wide and eighteen inches long. That is how paper towels were born. It was to take many years, however, before they gained acceptance and replaced cloth towels for kitchen use.
Production of the tissue paper
Tissue paper is produced on a paper machine that has a single large steam heated drying cylinder (yankee dryer) fitted with a hot air hood. The raw material is paper pulp. The yankee cylinder is sprayed with adhesives to make the paper stick. Creping is done by the yankee's “doctor blade” that is scraping the dry paper off the cylinder surface. The crinkle (creping) is controlled by the strength of the adhesive, geometry of the doctor blade, speed difference between the yankee and final section of the paper machine and paper pulp characteristics.
The highest water absorbing applications are produced with a through air drying (TAD) process. These papers contain high amounts of NBSK and CTMP. This gives a bulky paper with high wet tensile strength and good water holding capacity. The TAD process uses about twice the energy compared with conventional drying of paper.
The properties are controlled by pulp quality, creping and additives (both in base paper and as coating). The wet strength is often an important parameter for tissue paper.
Applications of tissue paper
Tissue paper has number of applications and they come in number of varieties such as hygienic tissues, facial tissues, paper towels, wrapping tissues, toilet tissues, table napkins etc.
- Facial tissue has been used for centuries in Japan, in the form of washi (??) or Japanese tissue, as described in this 17th-century European account of the voyage of Hasekura Tsunenaga. In 1924 facial tissue as it is known today was first introduced by Kimberly-Clark as Kleenex. It was invented as a means to remove cold cream. Kimberly-Clark also introduced pop-up, colored, printed, pocket, and 3-ply facial tissues.
- Paper towels are the second largest application for tissue paper in the consumer sector. This type of paper has usually a basis weight of 20 to 24 g/m2. Normally such paper towels are two-ply. This kind of tissue can be made from 100% chemical pulp to 100% recycled fibre or a combination of the two. Normally, some long fibre chemical pulp is included to improve strength. In 1951, William E. Corbin, Henry Chase (scientist), and Harold Titus began experimenting with paper towels in the Research and Development building of the Brown Company in Berlin, New Hampshire. By 1922, Corbin perfected their product and began mass-producing it at the Cascade Mill on the Berlin/Gorham line. This product was called Nibroc Paper Towels (Corbin spelled backwards). In 1931, the Scott Paper Company of Philadelphia, Pennsylvania introduced their paper towel for kitchens. They are now the leader of the manufacture of paper towels.
- W rapping tissue is a type of thin, translucent paper used for wrapping presents and cushioning fragile items.
- Table napkin, or face towel (also in Canada, the United Kingdom, Australia, New Zealand and South Africa: serviette) is a rectangle of cloth used at the table for wiping the mouth and fingers while eating. In the United Kingdom and Canada both terms, serviette and napkin, are used. In certain places, serviettes are those made of paper whereas napkins are made of cloth.
- Rolls of toilet paper have been available since the end of the 19th century. Today, more than 20 billion rolls of toilet tissue are used each year in Western Europe. Joseph Gayetty is widely credited with being the inventor of modern commercially available toilet paper in the United States.
Gayetty's paper, first introduced in 1857, was available as late as the 1920s. Gayetty's Medicated Paper was sold in packages of flat sheets, watermarked with the inventor's name. Seth Wheeler of Albany, New York, obtained the earliest United States patents for toilet paper and dispensers, the types of which eventually were in common usage in that country, in 1883. Moist toilet paper was first introduced in the United Kingdom by Andrex in the 1990s and in the United States by Kimberly-Clark in 2001 (in lieu of bidets which are rare in those countries).
With so many varieties available, tissue industry is growing leaps and bounds. Out of the world's estimated production of 21 million tonnes of tissue, Europe produces approximately 6 million tonnes tissue every year .
The European tissue market is worth approximately 10 billion Euros annually and is growing at a rate of around 3%. The European market represents around 23% of the global market. In North America, people are consuming around three times as much tissue as in Europe. In Europe, the industry is represented by The European Tissue Symposium (ETS), a trade association. The members of ETS represent the majority of tissue paper producers throughout Europe and about 90% of total European tissue production. ETS was founded in 1971 and is based in Brussels since 1992.
Finally, the paper tissue industry, along with the rest of the paper manufacturing sector, has worked hard to minimise its impact on the environment. Recovered fibres now represent some 46.5% of the paper industry’s raw materials. The industry relies heavily on biofuels (about 50% of its primary energy) and it is highly energy-efficient. EDANA, the trade body for the non-woven absorbent hygiene products industry (which includes products such as household wipes for use in the home) has reported annually on the industry’s environmental performance since 2005. The industry’s impact on the environment is in fact, relatively small.
All in all, the tissue sector continues to be a growth industry worldwide, offering new opportunities for companies to expand. Recently, there has been a great deal of interest, in particular, in the use of recovered fibres to manufacture new tissue paper products. However, whether this is actually better for the environment than using new fibres is open to question.
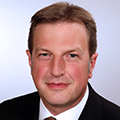
About: Devid - Head of Sales
Cras sit amet nibh libero, in gravida nulla. Nulla vel metus scelerisque ante sollicitudin. Cras purus odio, vestibulum in vulputate at, tempus viverra turpis. Fusce condimentum nunc ac nisi vulputate fringilla. Donec lacinia congue felis in faucibus.
Related Articles

Adhesives and Chemicals

Innovation of Bagasse in Pulp & Paper Industry
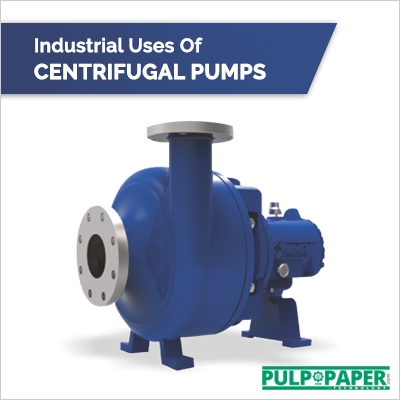
Industrial Uses Of Centrifugal Pumps
Top viewed articles, publish your article.
Thank you for your interest in publishing article with Packaging-Labelling. Our client success team member will get in touch with you shortly to take this ahead.
you're here, check out our informative and insightful article. Happy Surfing!
Client Success Team (CRM),

- Open access
- Published: 06 September 2022
Browning of the white adipose tissue regulation: new insights into nutritional and metabolic relevance in health and diseases
- Sabrina Azevedo Machado 1 na1 ,
- Gabriel Pasquarelli-do-Nascimento 1 na1 ,
- Debora Santos da Silva 1 ,
- Gabriel Ribeiro Farias 1 ,
- Igor de Oliveira Santos 1 ,
- Luana Borges Baptista 1 &
- Kelly Grace Magalhães ORCID: orcid.org/0000-0002-7435-5272 1
Nutrition & Metabolism volume 19 , Article number: 61 ( 2022 ) Cite this article
17k Accesses
50 Citations
46 Altmetric
Metrics details
Adipose tissues are dynamic tissues that play crucial physiological roles in maintaining health and homeostasis. Although white adipose tissue and brown adipose tissue are currently considered key endocrine organs, they differ functionally and morphologically. The existence of the beige or brite adipocytes, cells displaying intermediary characteristics between white and brown adipocytes, illustrates the plastic nature of the adipose tissue. These cells are generated through white adipose tissue browning, a process associated with augmented non-shivering thermogenesis and metabolic capacity. This process involves the upregulation of the uncoupling protein 1, a molecule that uncouples the respiratory chain from Adenosine triphosphate synthesis, producing heat. β-3 adrenergic receptor system is one important mediator of white adipose tissue browning, during cold exposure. Surprisingly, hyperthermia may also induce beige activation and white adipose tissue beiging. Physical exercising copes with increased levels of specific molecules, including Beta-Aminoisobutyric acid, irisin, and Fibroblast growth factor 21 (FGF21), which induce adipose tissue browning. FGF21 is a stress-responsive hormone that interacts with beta-klotho. The central roles played by hormones in the browning process highlight the relevance of the individual lifestyle, including circadian rhythm and diet. Circadian rhythm involves the sleep–wake cycle and is regulated by melatonin, a hormone associated with UCP1 level upregulation. In contrast to the pro-inflammatory and adipose tissue disrupting effects of the western diet, specific food items, including capsaicin and n-3 polyunsaturated fatty acids, and dietary interventions such as calorie restriction and intermittent fasting, favor white adipose tissue browning and metabolic efficiency. The intestinal microbiome has also been pictured as a key factor in regulating white tissue browning, as it modulates bile acid levels, important molecules for the thermogenic program activation. During embryogenesis, in which adipose tissue formation is affected by Bone morphogenetic proteins that regulate gene expression, the stimuli herein discussed influence an orchestra of gene expression regulators, including a plethora of transcription factors, and chromatin remodeling enzymes, and non-coding RNAs. Considering the detrimental effects of adipose tissue browning and the disparities between adipose tissue characteristics in mice and humans, further efforts will benefit a better understanding of adipose tissue plasticity biology and its applicability to managing the overwhelming burden of several chronic diseases.
The adipose tissues (ATs) are endocrine and dynamic organs that display high morphological and functional plasticity. White AT (WAT) was named that way because it presents white adipocytes in its composition. In contrast, brown AT (BAT) has as its main integrant the brown adipocytes [ 1 ]. Both ATs play various physiological roles, including energy storage, endocrine regulation, and thermogenesis. As a means of adapting, mammalians developed a mechanism to maintain their body temperatures under unfavorable climates [ 2 ]. This process, called adaptative thermogenesis, occurs due to the elevated plasticity of ATs, which allows reversible changes in their morphology and functions [ 3 ]. Studies have shown the capacity of progenitor cells as well as mature adipocytes to differentiate into a model that presents similarities with the brown profile, called brite or beige AT [ 4 , 5 , 6 ]. When this phenomenon occurs in mature adipocytes it is called browning of WAT [ 4 ].
During the browning process, WAT show increased mitochondrial number, augmented energy expenditure, fat multilocularization, and thermogenic genes expressions, such as Peroxisome proliferator-activated receptor-a (PPARα) and PPARγ, PR domain containing 16 (PRDM16), peroxisome proliferator-activated receptor-gamma coactivator 1 a (PGC1-α), cell death-inducing DNA fragmentation factor-like effector A (CIDEA), and Uncoupling Protein 1 (UCP1) [ 7 , 8 , 9 ]. WAT browning occurs in specific conditions through exposure to certain stimuli such as cold, thyroid hormones, diet, natriuretic peptides, medication, and exercise [ 10 , 11 , 12 , 13 , 14 ] (Fig. 1 ).
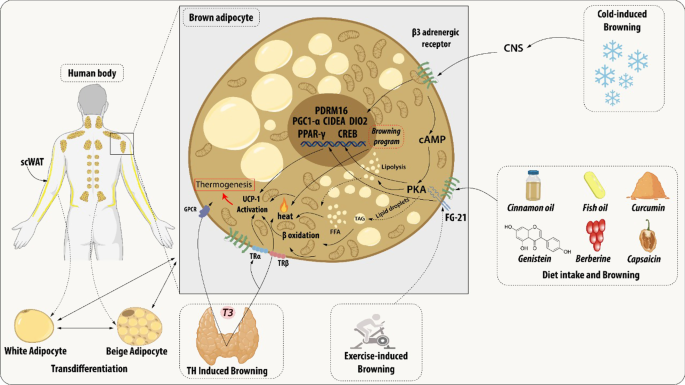
Brown adipocyte regulation by exogenous agents. Thyroid hormones act on thermogenesis through interaction with their Thyroid receptors (TR) and the G-protein-coupled receptor (GPCR). TRα promotes an increase in adrenergic signaling while TRβ acts to stimulate uncoupling protein 1 (UCP1). β3 receptor (β3-AR) is expressed constitutively on the surface of the adipocyte and acts to regulate the transcription and activation of genes related to mitochondrial biogenesis, brown adipocyte differentiation, and lipid storage. This receptor can be activated through cold, which is the main mechanism for activating browning, agonist drugs, or diet. Chronic exposure to cold and food intake, such as curcumin and fish oil, promotes thermogenesis by releasing catecholamines from the central nervous system (CNS) that bind to β-AR, thus initiating a signaling cascade. An increase in the concentration of cAMP is elicited which consequently leads to the enhanced activity of protein kinase A (PKA) which promotes the cAMP-response element-binding protein (CREB). This pathway is related to the transcription of thermogenic genes such as peroxisome proliferator-activated receptor γ (PPARγ), Type II iodothyronine deiodinase (DIO2), PR domain containing 16 (PRDM16), Peroxisome proliferator-activated receptor gamma coactivator 1-alpha (PGC-1α) and Cell death-inducing DNA fragmentation factor-like effector A (CIDEA). The other dietary components can participate in the induction of browning through the modulation of the gut microbiota promoting the increase of the expression of thermogenic genes. The release of growth factor 21 (FGF21) is mediated by the practice of physical exercises and physiological changes. FGF21 will interact with its FGFR receptor and that activation induces a self-phosphorylation of the FGFR that mediates the activation of pathways related to increased expression of UCP1
The increase in energy expenditure can act as a therapeutic approach to metabolic syndrome, and also can be associated with poor prognosis of diseases associated with hypermetabolism [ 15 , 16 , 17 , 18 ]. For this reason, efforts have been employed to identify the key participants in the regulation of browning. This review aims to present and describe the current studies related to both endogenous and exogenous most relevant agents and their biological mechanisms at biochemical and molecular levels.
Introduction
Originally cited as energy storage organs, exclusively, ATs are currently known to express and secrete a variety of bioactive peptides, the adipokines, including leptin, resistin, vaspin, visfatin, hepcidin, adiponectin, and inflammatory cytokines. These bioactive secreted factors act both locally and systematically, modulating different biological processes and consequently influencing the metabolism of various organs, such as the liver, muscle, pancreas, and brain via endocrine mechanisms [ 19 , 20 ]. Besides adipocytes, AT contains an extracellular matrix, nerve tissue, stromovascular cells, and immune cells, which together act as an integrated unit [ 21 ].
Presently, two main subtypes of ATs have been described: WAT and BAT. Brown and white adipocytes have widely different morphologies, not only in terms of composition but also in the form of lipid storage (number and size of lipid droplets) and the disposition and number of mitochondria. These differences correspond to distinct functional roles, diverging in energy metabolism, storage, and distribution [ 19 , 22 ].
WAT, the most abundant AT in the body, contains the white adipocytes, which present unilocular lipid droplets, scarce mitochondria, and lipid storage capacity [ 23 ]. Since the discovery of the adipokines, WAT is also recognized as an important endocrine organ, actively participating in the regulation of physiologic and pathologic processes, including immunity and inflammation [ 24 , 25 ]. Widely distributed throughout the body, there are two main representative types of WATs, the visceral WAT (vWAT) and the subcutaneous WAT (scWAT). While one is distributed around organs and provides protective padding, the other is located under the skin and provides insulation against heat or cold, respectively [ 26 ].
In contrast, brown adipocytes display multilocular lipid droplets, a large number of mitochondria, and thermogenic capacity due to elevated uncoupling protein 1 (UCP1) amounts anchored in its mitochondrial inner membrane [ 27 ]. The BAT utilizes this high mitochondrial content and elevated UCP1 amounts to uncouple oxidative phosphorylation from adenosine triphosphate (ATP) synthesis to dissipate chemical energy as heat [ 28 ]. Thus, BAT affects the metabolism of the entire body, being able to alter insulin sensitivity and modify the susceptibility to increase weight. For a long time, BAT was only considered an energy-producing organ in rodents and newborns, undergoing involution with age. However, BAT has also been identified in human adults near the aorta and within the supraclavicular region of the neck. Nevertheless, the origin of BAT is still under debate [ 26 , 29 ].
Recently, a type of AT showing intermediary characteristics between that of white and brown adipocytes, which has mixed structural features of both, was identified as beige AT [ 29 ]. This type of AT was reported as a set of adipocytes in WAT that might acquire a thermogenic phenotype with higher UCP1 expression, similar to brown adipocytes after enough stimulus [ 29 , 30 ].
There are two major mechanisms described related to beige cells arising: d e novo differentiation which occurs from a progenitor resident cell and transdifferentiation which consist of differentiation of a mature white adipocyte through a molecular mechanism. The first theory is based on that beige adipocytes come from progenitor cells differentiation induced by adipogenic stimulation such as cold exposure, adrenergic signaling, exercise, natriuretic peptides, thyroid hormones, diets, and food components [ 31 , 32 ]. Currently, several specific cell markers were identified in various types of progenitor cells such as in smooth muscle-like cells (Myh11 + ), preadipocytes (Pdgfrb + , SMA + ), adipocytes progenitor cells (Sca-1 + Pdgfra + CD81 + ) [ 33 , 34 , 35 ]. These adipogenic stimulation actives transcriptional machinery of browning that is characterized by the expression of Ucp1, Prdm16, Zfp516, and Pgc1a genes that will promote a beige differentiation [ 36 ].
On the other hand, the transdifferentiation hypothesis proposes beige cells arise from mature white adipocytes, in a reversible process, after adipogenic stimulus without the participation of a progenitor-like state of cells [ 37 ]. The underlying molecular mechanisms for transdifferentiation are under intensive research, but some studies already show that this plasticity process occurs mainly in scWAT depots [ 29 ]. Known as browning, this process has gained increasing attention in the research area as an alternative method of energy stimulation. UCP1 expression can be stimulated when white adipocytes are exposed to stimuli, previously referred as to adipogenic stimulus, [ 20 , 27 , 29 ], driven by a set of molecules known as browning markers.
The uncoupling protein 1 (UCP-1)
The non-shivering thermogenesis is a phenomenon that occurs in brown and beige ATs due mostly to the action of UCP1 [ 38 ]. UCPs are transmembrane proteins that belong to the mitochondrial anion carrier family (MACF), i.e., mediate specific metabolite exchanges between the cell cytoplasm and the mitochondrial matrix and thus enable the activation of essential biochemical pathways [ 39 , 40 ]. The UCPs exhibit 5 isoforms, ranging from UCP1 to UCP5 are present in several tissues [ 41 , 42 ]. UCP1 is the main isoform associated with thermogenesis, it is widely and selectively expressed in the inner mitochondrial membrane of the adipocyte, representing about 10% of the total mitochondrial protein in human epicardial AT [ 43 , 44 , 45 , 46 ].
UCP1 protein is described as participating in thermogenesis by interfering in proton leakage within the chemiosmotic gradient during the mitochondrial oxidative phosphorylation by the translocating fatty acids (FAs). This gradient is obtained from the oxidation of substrates and provides the required force to induce the respiratory machinery to produce ATP. Once UCP1 promotes proton leakage, the energy obtained cannot be stored in the form of ATP and is alternatively dissipated as heat [ 47 , 48 ]. Thus, it is evident that direct regulation of UCP1 protein activity is one of the means of regulating thermogenesis, and that occurs in opposite ways by cytosolic purine nucleotides and long-chain fatty acids (LCFA), promoting inhibition or activation of UCP1, respectively [ 49 ].
The other form of regulating UCP1 is at the transcriptional level. UCP1 gene is transcribed only in brown and beige adipocytes, associates with the differentiation state of these cells, and is quantitatively regulated in response to many physiological signals [ 9 ]. These characteristics are consequences of the transcriptional control mediated by trans-acting factors on regulatory regions found in the 5’ non-coding region of the UCP1 gene. The proximal regulatory region, which is found immediately upstream of the transcription start site, contains cAMP response element-binding protein (CREB) [ 50 , 51 ] and CCAAT-enhancer-binding protein (C/EBP) [ 52 ] binding sites. Also in the proximity of the site of transcription start, activating transcription factor-2 (ATF2)-binding site interacts with transcriptional coregulators, such as PGC-1α, impacting UCP1 gene transcription [ 9 ]. In opposition to these proximal regulatory sites, a strong enhancer region is placed more than 2 kb upstream of the transcription initiation site [ 50 , 53 ] and contains a cluster of response elements for nuclear hormone receptors [ 7 , 54 ].
UCP1 gene activation and repression depend on which trans-acting factors bind to the regulatory region. For example, CREB binding sites mediate a positive transcriptional response to cAMP [ 50 ] and a negative response to AP2 (c-Jun/c- Fos) complexes [ 51 ]. Another example is the PPARγ binding site found in the distal enhancer region, which associates with gene activation after binding to its main ligand but represses UCP1 transcription when interacting with liver X receptor (LXR) and its corepressor receptor-interacting protein 140 (RIP140) [ 55 ]. RIP140 inhibits UCP1 gene transcription by enabling the assembly of DNA and histone methyltransferases on the UCP1 gene, altering the methylation status of CpG islands in the promoter region and histones, impacting gene expression through transcription machinery accessibility [ 56 ].
Although some epigenetic modifications are associated with repressed UCP1 gene expression, as in H3K9 demethylation marks, chromatin modifications indicative of activation of this gene also occur, such as in the case of H3K4 trimethylated marks, which are enriched in BAT [ 57 ]. Also participating in fine-tuning of gene expression, microRNAs (miRs) are characterized to be a group of short non-coding RNAs (ncRNAs) generated by the sequential processing of longer ribonucleic acid molecules [ 58 ]. While miR-328 [ 59 ] and miR-455 [ 60 ] are described to be activators of UCP1 gene expression, miR-27 [ 61 , 62 ], and miR-133 [ 63 ] display UCP1 gene transcription inhibitory activity .
The roles of WAT and BAT in metabolic syndrome is well characterized, but the physiological and biochemical modulations of BAT remain unclear [ 64 , 65 , 66 ]. Several studies showed that UCP1-dependent BAT activity was mostly found to be beneficial in decreasing inflammation, and improving cardiometabolic homeostasis [ 67 , 68 , 69 ]. However, this tissue has a lower activity in obese in comparison to healthy individuals [ 70 ].
It is well established that the deficiency of the UCP1 gene is not enough to protect against diet-induced obesity (DIO), but can modulate important physiological and metabolic parameters in mice [ 64 , 65 ]. The food intake-induced browning is inhibited in the absence of UCP1, demonstrating the intimate relationship between this differentiation process and UCP1 [ 71 , 72 ]. More than that, UCP1 -/- female mice fed with a western diet displayed increased whitening of BAT, as well as metabolic disruption indicated by glucose intolerance, upregulation of genes related to inflammation, liver steatosis, immune cell infiltration, and endoplasmic reticulum (ER)/oxidative stress [ 73 ]. Accordingly, even during standard diet under cold exposure, UCP1−/−, male mice showed BAT immune cell infiltration and ER stress profile [ 74 ]. The lack of UCP1 promotes de novo lipogenesis and hyperplasia of inguinal WAT, leading to an increase in FA trafficking to the liver [ 75 ].
In contrast, the upregulation of UCP1 or even only its activation can perform a paradoxical role in hypermetabolic scenarios and associate with a worse prognosis [ 76 , 77 ]. It is proven that diet-induced whitening is related to the upregulation of this gene. A greater expression of browning markers (e.g., UCP1, PGC-1α, TBX1) was found in obese human patients mainly in vWAT [ 78 ] Besides that, mice affected by cancer-associated cachexia tend to show increased thermogenesis gene expression and BAT activation [ 79 ].
The browning process is spontaneously induced by tumor-secreted factors and IL-6 during cachexia development, which can lead to full depletion of AT [ 80 , 81 ]. Interestingly, the elicitation of browning after burn injury is associated with the hypermetabolic response, as well as an increase in lipolysis and free fatty acid efflux that can outcome in liver steatosis [ 82 , 83 ]. In addition to the therapeutic impact of the browning process in obesity and metabolic diseases, recent discoveries regarding the impact of UCP1-dependent BAT activity in hypermetabolism conditions should be further investigated in the context of UCP1 to appropriately regulate browning for application in different situations.
Beta 3 adrenergic receptor activation
Increasing energy expenditure through activation of BAT shows potential for treating metabolic diseases, and that is the reason this approach has been deeply investigated [ 84 ]. The white/brown plasticity of ATs and tissue thermogenesis appear to be activated by a β-3 adrenergic receptor (β-3AR) system [ 4 , 30 , 85 ]. β3-ARs are expressed predominantly on white and brown adipocytes [ 86 ]. Murine WAT expresses β3-AR transcripts in a greater proportion compared to other β-ARs, similar to BAT [ 87 ]. Although β3-AR mRNA levels are lower in humans than in rodent AT, its roles seem to be fundamental in the regulation of energy balance and glucose homeostasis [ 88 ].
Browning of WAT occurs mainly by noradrenaline and adrenaline stimulation, which influence lipolysis after binding to different adrenoceptor subtypes on the cell-surface membrane of fat cells. The interaction with β3-AR initiates a cascade of signal transduction that ends with the overexpression of thermogenic proteins, such as UCP-1 [ 88 , 89 ]. The adaptive thermogenic response is initiated by the central (CNS) and sympathetic (SNS) nervous systems with the release of norepinephrine (NE) and stimulation of β3-AR, through the G protein-coupled receptor Gs, which in turn activates the adenylyl cyclase (AC), stimulating the production of cyclic adenosine monophosphate (cAMP), and activating the protein kinase A (PKA) pathway. Then, these signals from the cAMP pathway, finally, upregulate UCP-1 and lipolysis [ 88 , 89 , 90 , 91 , 92 ].
A distinguishing feature of the β3-AR, already seen in past studies, is that it appears to be relatively resistant to desensitization and down-regulation, leading to the hypothesis that one of its functions might be to maintain signaling during periods of sustained sympathetic stimulation, as in diet-associated β3-AR activation or cold exposure [ 87 ]. Cold temperature exposure elicits a coordinated physiological response aimed at maintaining their body temperature. This response activates the mentioned cascade and generates heat in beige adipocytes within scWAT and BAT [ 85 ]. Thus, it was seen that mice with a combined target disruption of the three β1, β2, and β3 adrenergic receptors (TKO mice) have increased susceptibility to cold-induced hypothermia as well as diet-induced obesity [ 91 ]. Thereby, mice β3-AR activation started to be studied, effectively mimicking cold exposure effects [ 84 , 91 ].
Initial studies demonstrated that WAT UCP1 mRNA and protein levels are strongly decreased in β3-AR knockout (KO) mice [ 30 , 93 ]. In addition, β3-AR agonists are well-known for inducing ectopic UCP1 expression in WAT coupled with a significant mitochondrial enhancement in rodents, and for augmenting glucose homeostatic activity of their BAT [ 84 , 94 ]. On the other hand, in humans, early efforts to increase browning activation with the use of β3-adrenoreceptor agonists have failed in clinical trials because of their β1- and β2-AR-mediated cardiovascular effects [ 13 , 84 , 94 ]. However, a recent study showed that mirabegron, a selective β3-agonist previously developed for the treatment of overactive bladder, was shown to increase BAT activity as compared to placebo. This study used an oral dose of 200 mg in healthy male subjects, and despite not having severe cardiovascular side effects, they have been shown to increase heart rate and systolic blood pressure [ 84 ]. That is the reason long-term studies are warranted to investigate the effectiveness and cardiovascular safety of this type of treatment to induce weight loss and metabolic health improvements.
Genetic factors must be considered in influencing adipocyte lipolysis regulation. Genetic variance in β3-AR and its specific G-coupling protein has functional effects on lipolysis. Polymorphism in the G-β3 gene, for example, influences catecholamine-induced lipolysis in human fat cells by altering the coupling of β3-AR to G-proteins [ 88 ]. This proves once again the importance of the β3-AR presence for the thermogenic process.
Temperature-induced browning
It is well established that temperature can modulate biochemical, inflammatory, and immunological processes systemically, displaying relevant physiological impact [ 95 , 96 ]. Despite this, the influence of warmer temperatures is better described compared to cold conditions due to their immediate danger. Fever, triggered by infectious and inflammatory processes, was associated with a worse prognosis in the past centuries, demanding greater medical attention for a long time [ 97 ]. However, currently, it is recognized that both hyper and hypothermia, in properly regulated circumstances, are beneficial response mechanisms to infection in mild and severe profiles, respectively [ 98 ].
Hypothermia is also associated with an advantageous mechanism against severe systemic inflammation. In experimental studies, the infectious or aseptic systemic inflammation process is elicited by the intravenous administration of bacterial lipopolysaccharide (LPS) in mice [ 99 , 100 , 101 ]. The variances of body temperature are modulated by the environmental temperature and concentration of LPS introduced [ 102 ]. Animals housed in hyperthermal conditions or exposed to lower LPS concentrations displayed polyphasic fever. In thermoneutral conditions, the fever was also usually elicited to induce the immunological response. However, if the mice were housed in cooler acclimation or administered with higher LPS concentrations, the effect elicited was hypothermia, which associates with arterial hypotension aimed to avoid infection spread, followed by polyphasic fever.
The ideal body temperature is obtained by the modulation of blood vessel tension degree, and heat production by thermogenesis. Cutaneous vasoconstriction and thermogenesis processes occur to increase body temperature and avoid heat loss. Conversely, the opposite effect, skin vasodilatation and thermogenesis inhibition, is stimulated to induce hypothermia [ 102 , 103 , 104 , 105 ].
Temperature is a paradoxical agent with important roles not only in biological events but also in the development of several diseases [ 106 , 107 , 108 , 109 , 110 ]. Hypothermia, specifically, displays a typical profile, in which the energy is preserved. The decrease in body temperature also favors the development of an anti-inflammatory profile and immunosuppression, which can act as a double-edged sword depending on the condition [ 108 , 111 , 112 , 113 ]. In the tumoral context, hypothermia provides an immunosuppressive, hence, pro-tumoral microenvironmental due to impaired CD8+ T cell function, an increase of the regulatory and Th2 cells, and higher levels of the cytokines IL-4 and IL-10, which increase cancer progression and metastasis [ 114 , 115 , 116 ].On the other hand, hyperthermia is recognized to elicit a more robust immune response against infection, injury, and cancer [ 117 ]. Several studies reported an increase in IFN-γ and IL-2 secretion from peripheric T cells, enhancement of cytotoxicity, DC maturation, and increase of tumor-specific CD8+ T cells [ 118 , 119 , 120 , 121 ].
In inflammatory conditions, such as neurological damage, atherosclerosis, systemic inflammation, and hypothermia (cryotherapy) can be beneficial [ 122 , 123 , 124 , 125 , 126 ]. Cryotherapy benefits are illustrated by an experimental approach that submitted healthy and physical activity practitioners men to intense exercises aimed to induce muscle injury [ 127 ]. It was observed that cryotherapy mediated the increase of IL-10, reduction of pro-inflammatory cytokine IL-1, reduction of muscle damage and blood cholesterol, decrease oxidative stress and improve the lipid profile not only in healthy patients but also in patients with active-phase ankylosing spondylitis [ 124 , 128 , 129 ]. Cryotherapy also shows to be neuroprotective capacity, alleviating sequelae from ischemic or hemorrhage stroke, cardiac arrest, intracranial pressure elevation, and traumatic brain injury [ 130 ].
In the same line, recent research evaluated the impact of spontaneous body hyperthermia after brain injury. Metabolic modulations were observed as the diminishment of both cerebral and arterial glucose levels and increase of lactate-pyruvate ratio. However, these changes were not associated with a worse prognostic [ 131 ]. Additionally, induced hyperthermia in healthy men promoted an increase in cerebral metabolic rate of oxygen (CMRO 2 ), also increase IL-6 and myeloperoxidase (MPO) systemically, but did not promote the same inflammatory and oxidative phenomenon in the brain [ 132 ]. In peripheral organs such as the liver, hyperthermia is associated with an increase in oxidative metabolism, vasodilation, and an increase of heat shock proteins (HSP) expression. HSP displays an important role in metabolism such as modulation of both glucose and lipid metabolism in the liver and improving the mitochondrial skeletal muscle functionality [ 133 ].
In addition, temperature modulates directly the shivering and non-shivering thermogenesis processes. The occurrence of these events maintains proper body temperature under adverse thermal acclimation. Once shivering thermogenesis is decreased in cold acclimation (around 4 °C), non-shivering thermogenesis is the major way to produce heat in this context [ 2 , 4 ]. The detection of the thermal changes begins with the capture of sensory stimuli by cutaneous thermoreceptors, which promote the sensitization of afferent nerves. The stimuli are directed to the CNS, which then induces thermoregulatory responses, including vasoconstriction and catecholamines secretion. These catecholamines, mainly NE, increase BAT activation, hence heat production through a UCP1-dependent manner [ 134 , 135 ].
BAT is a highly innervated and vascularized organ that displays considerable amounts of β3-ARs, which is also expressed in WAT, though at a lower level. NE binding to β3-ARs promotes systemic adrenergic activation, which induces a signal cascade culminating in the accumulation of adipokines, such as Zinc-α2-glycoprotein (ZAG), increase in thermogenesis-related gene expression, as UCP1, thus enabling mobilization and oxidation of free fatty acids (FFAs) in both tissues, increasing BAT activity and promoting browning in WAT. [ 84 , 136 , 137 ].
It is known that the results observed in humans do not always represent the same effects previously described in mice or even contradictory results can be obtained under similar conditions for the same species [ 138 ]. Unfortunately, this premise can also be applied to cold-induced browning. Leitner and colleagues showed that in human fewer than half of the BAT deposits is stimulated by cold exposure, hence, the thermogenic function was lower than expected [ 44 ].
Brychta and others demonstrated that the profile of men with obesity was associated with a reduced tolerance limit to chill temperatures, suggesting that thermogenesis was diminished in these individuals, as well as energy expenditure [ 139 ]. Blauw et al. [ 140 ] demonstrated a surprising association between the impairment of glycemic homeostasis, diabetes, and obesity in humans housed in the United States of America (USA) at warmer temperatures and associated these results with a decrease in BAT activity. Taking the assessment on a global scale, Kanazawa evaluated the parallel between higher temperatures, weight gain, and obesity. The data analyzed allowed to predict that global warming could be responsible for an increase of more than 10% in the obesity rates in 120 years, counting from 1961 [ 141 ].
Notably, the use of cold as a browning inducer has been carefully applied not only because of the side effects that can be displayed at the whole-body level but also due to the contradictory effect observed in humans. If on one hand, the anti-inflammatory and immunosuppressive role mediated by cold and cold-induced browning is beneficial in healthy individuals, for ill people these same effects may become harmful. In contrast, intriguing research has brought a new perspective on temperature-based browning. Li and colleagues discovered that a hydrogel-based photothermal therapy leads to a successful increase of beige activation in both mice and humans. The therapy consists of increasing the local temperature, around 41˚C, without evident stress on skin or adjacent tissues [ 142 ]. This promising study succeeds previous findings that pointed to the occurrence of WAT browning after burn injury [ 143 , 144 ]. The characterization of possible inducers of browning is a strongly growing field since the applicability of these inducers as therapy in humans has proven to be a major clinical hurdle. However, even under promising advances is clear that further investigation regarding the mechanisms triggered by this stimulus pathway should be conducted.
Exercise-induced browning
Physical exercising is already associated with improvements in several processes related to the cardiovascular system, skeletal muscle, and ATs [ 193 ]. Following this, several studies show that physical activity provides better quality of life [ 194 ] and helps in the treatment of several metabolic diseases and obesity [ 195 ] through increasing AT lipolysis, vascularization, blood flow, and promoting the secretion of hormones and adipokines [ 196 ]. Among the main adipokines, FGF21 (Fibroblast growth factor 21) and leptin stand out, which act in an autocrine/paracrine manner, regulating WAT browning process [ 197 ]. After physical activities, the adipokine leptin stimulates activity in the sympathetic nerve and together with insulin act synergistically in different neuronal subsets of proopiomelanocortin (POMC) inducing browning of WAT through decreased hypothalamic inflammation caused by exercise [ 198 ]. During exercise, the increase in glucagon, which already has thermogenic potential [ 199 ], and the decrease in insulin in the liver lead to FGF21 secretion [ 200 ].
The exercise induces pleiotropic effects in the liver, AT, immune system, and skeletal muscle by enabling myokine secretion upon contraction [ 201 ]. After activities, muscle cells increase the expression of PGC-1α, inducing BAT thermogenesis and mitochondrial biogenesis. Among the myokines involved in the browning process, interleukin-6 (IL-6) is a modulatory cytokine secreted by several tissues, including skeletal muscle and AT. A study showed that mouse AT when treated with IL-6 for 6 h induces the expression of PGC-1α and mitochondrial enzymes [ 202 ]. In addition, analyses showed that IL-6 is involved in the increase of UCP1 mRNA in inguinal WAT (igWAT) stimulated by physical activity [ 203 ]. Another relevant myokine is Irisin; Once exercising increases the expression of PGC-1α, it induces increased levels of the fibronectin domain-containing protein 5 (FNDC5) protein, which, after being cleaved, is released as the hormone irisin [ 204 ]. Irisin was shown to stimulate UCP1 expression and thermogenic differentiation of white fat precursor cells in vitro and in vivo [ 145 ].
The myokine myostatin (Mstn), a growth factor that limits muscle growth and development, is negatively involved in the WAT browning process, as Mstn-deficient mice showed high expression of genes associated with FA oxidation, mitochondrial biogenesis, lipid transport together with the positive regulation of PGC-1α and UCP1, this mechanism occurs through the phosphorylation of AMPK, necessary for the activation of PGC1α and FNDC5 [ 199 ]. Metrnl, the gene encoding for Meteorin-like protein, is a myokine known to be induced by resistance exercise dependently on PGC-1α4. Metrnl regulates genes involved in thermogenesis, as it is capable of promoting the activation of M2 macrophages by inciting the expression of IL-4 and thus triggering the production of catecholamines [ 206 ], responsible for favoring thermogenesis in AT [ 207 ]. Beta-Aminoisobutyric acid is another myokine that has increased levels during exercise and can induce the brown adipocyte phenotype in human-induced pluripotent stem cells during differentiation to mature white adipocytes [ 146 ].
Intense physical activity causes increased heart rate and stretching of cardiomyocytes, which cause the secretion of atrial natriuretic peptide (ANP) and brain natriuretic peptide (BNP), molecules that stimulate lipolysis, UCP1 expression, and mitochondrial biogenesis [ 208 ]. It also induces an increase in lactate, which binds to receptor GRP81 on adipocytes, leads to an increase in P38 phosphorylation, and thus mediates the browning of WAT by activating the PGC-1a, PPAR, γ, and Ucp1 genes [ 147 ]. During lipolysis, FAs are not only used as an energy source but also undergo the re-esterification process where they are converted into triglycerides in AT. This re-esterification consumes ATP generating AMP. AMP in turn can activate AMPK, which then induces greater expression of PGC-1α and mitochondrial biogenesis [ 210 ].Another the important effect induced by exercise that plays an important role in the browning of WAT is oxidative stress in skeletal muscle, whish it responsible for the increase in H 2 O 2 through the reduction of glutathione levels, a molecule capable of supplying electrons to glutathione peroxidase, thus increasing H 2 O 2 levels. And also by increasing the activity of superoxide dismutase 2 (SOD2), which reduces ROS to H 2 O 2 . When H 2 O 2 enters the circulation, it is directed to WAT and subsequently induces the expression of thermogenic genes [ 148 ]. Exercise also increases the level of succinate, resulting in augmented levels of mitochondrial reactive oxygen species, which in turn promotes the sulphenylation of Cys253 to increase UCP1 activity [ 149 ].
Although the studies conducted in mice seem promising, the effect of exercise on WAT browning in humans has proven to be controversial. A survey conducted with sedentary subjects participating in a 12-week bicycle-training program showed scWAT increased expression of UCP1, carnitine palmitoyltransferase 1B (CPT1B), TBX1 [ 15 ]. However, other studies have not achieved similar effects. Tsiloulis and colleagues collected scWAT of obese men after 6 weeks of physical training and the mRNA levels of UCP1, CD137, CITED, TBX1, LHX8, and TCF21 were not altered [ 211 ]. Many factors may be involved in this diversity of results since the duration, frequency, and degree of intensity are associated with these effects. Thus, more human studies need to be conducted as many questions still need to be clarified.
Fibroblast growth factor 21
The fibroblast growth factor family (FGF) performs a range of cellular metabolic and physiological responses to maintain overall homeostasis. FGF 21 was first identified in mice and humans in 2,000 by Nishimura and colleagues through cDNA identification in different organs [ 150 ]. While the gene in mice is located in chromosome 7 and encodes a preprotein of 210 amino acids (aa), in humans it is found in chromosome 19 and encodes a preprotein of 209 aa. Proteins belonging to the FGF family exert a wide range of functions, from promoting cell proliferation and differentiation to systemic effects [ 151 , 152 ], acting as autocrine/paracrine/endocrine factors [ 153 ]. Most FGF family members have a high affinity to heparin sulfate, except for the endocrine FGF (FGF) subgroup, which consists of FGF 19 (FGF 15 in rodents), FGF 21 e FGF 23 in humans [ 151 ]. FGF molecules lack an extracellular heparin-binding domain and thus can enter the blood system [ 154 ].
FGF 21 binds to a fibroblast growth factor tyrosine kinase receptor (FGFR), which can be found in seven isoforms: 1b, 1c, 2b, 2c, 3b, 3c, and 4. The FGF 21 requires its dimerization with a klotho protein, called beta-klotho (KLB). Thus, the FGFR-KLB receptors lead to the intracellular cascade that goes through the phosphorylation of FGFR substrate 2α (FRS2α) and the activation of Ras-MAPKs and PI3K-Akt kinases [ 154 , 155 , 156 ]. Once FGF21 signaling requires KLB to activate FGFRs, the co-expression of these two receptors determines the sensitivity of a tissue or organ to FGF21 [ 157 ]. FGF 21 is defined as a stress-responsive hormone [ 152 ], which effect is subtle in physiological conditions but significantly exacerbated under nutritional, metabolic, oxidative, hormonal, or environmental challenges. Consequently, starvation and overfeeding, ketogenic and high-carbohydrate diets, physical exercises, protein restriction [ 158 ], type 2 diabetes (T2D), obesity [ 153 ], and nonalcoholic fatty liver disease (NAFLD) [ 159 ] can induce the expression or/and signaling of FGF 21 [ 158 ].
FGF 21 is synthesized mainly in the liver and thymus but is also detected in skeletal muscle, pancreas, intestine, heart, β cells, and WAT and BAT [ 160 ]. As an important metabolic regulator, acting mostly in glucose and lipid homeostasis, FGF 21 triggers lipolysis and FFAs released in circulation from WAT during prolonged fasting or starvation [ 29 , 160 ]. PPAR-α is activated in the presence of FFA and improves FFA oxidation and ketone bodies formation for acting as energy sources during prolonged fasting. The interaction between FFA/PPAR-α/retinoid X receptor (RXR) has a PPAR-α response element, which activates FGF 21 promoter [ 161 , 162 ]. Thus, when PPAR-α activity increases, the production of FGF 21 in the liver also augments, leading to energy production, increased ketogenesis, gluconeogenesis, appetite, and systemic glucose uptake as adaptive responses to starvation [ 163 ]. The activity of FGF 21 is not limited to starvation conditions, but it is also increased in adaptation to high-fat (HF) intake [ 164 ].
Human studies inform that FGF21 production is stimulated in situations of decreased thermogenesis, reduction in adiponectin levels, and tissue breakdown markers, such as transaminases elevation mare than changes in levels of FFAs [ 165 ]. Another means of increasing FGF21 levels, through PPAR-α activity, is through intense physical activity, growth hormone therapy, lactation, and milk ingestion in neonates [ 163 , 166 ]. Macronutrients such as proteins also regulate FGF 21 production through amino acid restriction [ 167 ]. This process starts when the general control non-derepressible 2 (GCN2)-eukaryotic initiation factor 2 (eIF2) α pathway is activated inducing the binding of activating transcription factor 4 (ATF4) to PGC-1 α [ 168 , 169 ].
After being secreted, its most important target is WAT, where FGF21 improves insulin sensitivity [ 153 , 160 ] and increments GLUT1 expression and consequently glucose uptake, as shown by in vitro 3T3-L1 adipocyte analyses [ 153 , 170 ]. The response element-binding protein (ChREBP) is sensitive to carbohydrates in the liver and ChREBP interaction with PPAR-γ in adipocytes modulates the expression of FGF21. In other words, the upregulation of ChREBP may induce the expression of this FGF [ 160 ]. Another example of FGF 21 influence on carbohydrate metabolism is through the suppression of hepatic pyruvate dehydrogenase (PD) complex through PD kinase 4 activity [ 171 ]. Additional transcription factors, such as retinoic acid (RA) receptor β (RARβ), TRβ, cyclic AMP response element-binding protein H (CREBH), RA receptor-related orphan receptor α (RORα), respond to determinants in the liver and regulates FGF 21 production [ 151 ].
WAT is not only a target of FGF21, but it is the major mediator of its effects. The processes of glucose- and insulin-sensitive responses depend on adiponectin production and secretion by this tissue [ 172 ]. Adiponectin also reduces the levels of sphingolipid ceramides in obese animals, which have been associated with lipotoxicity [ 173 ]. The action of FGF21 in WAT includes paracrine and autocrine actions and is mediated through the induction of PGC-1α protein in cold and through the enhanced levels of the thermogenic protein UCP1, which is a key protein for heat production [ 174 ]. BAT requires the FGFR1/KLB complex to respond to FGF21, which induces glucose uptake and thermogenesis through the induction of UCP1, in response to its autocrine and paracrine production. FGF 21 impact derives from increased PGC-1α levels and, consequently, expression of UCP1 [ 174 ]. In conclusion, FGF 21 is involved in glucose uptake, lipogenesis, and lipolysis, depending on the metabolic state of the adipocytes. This dual phenomenon may depend on nutritional condition, FGF21 concentrations reached between pharmacological administration and physiological secretion [ 175 ].
Thyroid-hormone-induced browning
Thyroid Hormone (TH) is essential for metabolism in mammals and associates with many processes, including organism development, metabolic regulation, neural differentiation, and growth [ 176 ]. Many genes are regulated after its conversion from the prohormone thyroxine (T4) to the activated form triiodothyronine (T3) [ 177 ] by 5′-deiodinase type 2 (D2), enzyme known to be expressed in the hypothalamus, WAT, BAT, and skeletal muscle, and to be required for adaptive thermogenesis [ 178 ]. TH is produced in the follicles of the thyroid gland and is synthesized through iodination of tyrosine residues in the glycoprotein thyroglobulin [ 179 , 180 ]. The main means of regulator its production is through thyroid-stimulating hormone (TSH), which binds to the TSH receptor (TSH-R) expressed in the thyroid follicular cell basolateral membrane and is released by the anterior pituitary in response to a circulating TH [ 181 ].
The biological response of TH is complex and highly regulated. It is mediated by thyroid hormone nuclear receptors (TRs). The TR genes produce two main types of receptors, α and β, and their isoforms α1, α2, α3, β1, β2, and β3, but only α1, β1, β2, and β3 are T3-binding receptors, which are differentially expressed in tissues and have distinct roles in TH signaling [ 178 , 182 ]. TH enters the cell through membrane proteins monocarboxylate transporter 8 (MCT8) and solute carrier organic anion transporter family member 1C1 9 (OATP1C1), then interacts with TR in the nucleus, which binds to the genomic thyroid-hormone responsive elements (TREs) and other nuclear proteins, including corepressors, coactivators, and cointegrators, leading to chromatin remodeling and the regulation of the UCP1 gene transcription [ 183 , 184 ].
This hormone is correlated with weight and energy expenditure. Thus, hypothyroidism, characterized by diminished TH levels, leads to hypometabolism, a condition associated with reduced resting energy expenditure, weight gain, high cholesterol levels, reduced lipolysis, and gluconeogenesis. On the other hand, hyperthyroidism, and elevated TH levels, induce a hypermetabolic state, characterized by increased resting energy expenditure, lower cholesterol levels, increased lipolysis and gluconeogenesis, and weight loss. Consequently, TH controls energy balance by regulating energy storage and expenditure regulating key metabolic pathways [ 178 ].
TH regulates basal metabolic rate (BMR) through ATP production, used for metabolic processes, and by generating and maintaining ion gradient [ 185 , 186 , 187 ]. BMR is induced by the stimulation of two main gradients, the Na+/K+ gradient across the cell membrane and the Ca 2+ gradient between the cytoplasm and sarcoplasmic reticulum and produces heat during ATP hydrolysis [ 188 ]. TH maintains the BMR levels through the uncoupling oxidative phosphorylation in the mitochondria. When ATP production is compromised in skeletal muscle, TH increases the leak of protons through the mitochondrial inner membrane, stimulating more oxidation to maintain ATP synthesis [ 189 ].
TH regulates metabolism primarily through actions in the brain, WAT, BAT, skeletal muscle, liver, and pancreas [ 178 ]. This action, as already said, is through TH receptors (TR) isoforms, WAT has the adrenergic signaling increased by TRα [ 190 ], otherwise BAT expresses TR α and β, as it needs TRα for adrenergic stimulation and TRβ for stimulating of UCP1, both for thermogenesis [ 176 ]. In humans, T3 administration induced UCP1 expression, dependent on the presence of TRβ, which induces “browning” [ 191 ]. TH regulates several aspects of lipid metabolism and human BAT from lipogenesis to lipoprotein signaling [ 192 ]. Rats administrated with T3 showed how the central nervous system is important to the activation of BAT by TH through inhibition of hypothalamic AMP-activated protein kinase (AMPK). Stimulation of sympathetic nervous system (SNS) activity leads to thermogenic gene expression in BAT [ 193 ].
As discussed previously, β-AR is stimulated by NE in response to SNS [ 1 ]. The expression of UCP1, required for BAT thermogenesis, is regulated by NE and T3 synergistically, once the induction in separate is twofold, while combined is 20 -fold [ 194 ]. Another way that UCP1 expression and thermogenesis are induced is through bile acid stimulation. G protein-coupled membrane bile acid receptor (TGR5) is stimulated in BAT and results in D2 stimulation and local T3 production [ 178 ].
In conclusion, several mechanisms have been proposed for the TH influence in the browning process, including cold exposure, adrenergic activation [ 167 ], and bile acid signal [ 178 ]. Thus, the stimulation of BAT activation and WAT browning increase the energy expenditure, loss of weight [ 195 ], D2 activation, UCP1 level increase, and consequent thermogenesis [ 192 ].
Circadian rhythm and browning
As previously discussed here, several exogenous factors are able to elicit browning of WAT and BAT activation. However, endogenous factors also play an important role in regulating the phenotype and physiology of these tissues. One of the most important endogenous factors that are related to the regulation of AT is the circadian rhythm, which is a refined system that acts as a master biological clock synchronizing daily and seasonal variations with the behavioral, cellular and tissue-autonomous clock, as well as several biological processes that include sleep–wake cycle, hormone secretion, lipid and glucose homeostasis, energy balance and body temperature [ 196 ]. The circadian rhythm is controlled by melatonin synthesis, which can occur both in the CNS, more specifically in the pineal gland being regulated by light/dark stimulus via the suprachiasmatic nucleus of the hypothalamus (SCN), and in peripheral tissues where its regulation remains unclear [ 197 ].
Disruption of circadian rhythm caused by aging, shift-work, irregular sleep, insomnia, or long exposure to light during the night is associated with sleep and metabolic disorders such as cardiovascular diseases, diabetes type 2 and obesity. Regarding metabolic diseases, AT plays a central role in metabolic and whole-body energy homeostasis, once its secretes several adipokines that regulate diverse processes in CNS and peripheral tissues. Leptin, a hormone mainly produced by adipocytes, is released into the circulation where it crosses the blood–brain barrier (BBB), through a saturable system, and interact with its receptor in the hypothalamus LepRb [ 198 , 199 ]. Hsuchou and colleagues demonstrated that leptin signaling disruption through a pan-leptin receptor knockout (POKO) in mice was able to dysregulate feeding behavior, metabolic and circadian rhythm profile and thus promote an accentuating of obesity [ 200 ]. Beyond control of feeding and metabolic processes, leptin also displays a role in energy balance through the increase of AT thermogenesis in BAT by sympathetic activation [ 201 , 202 ].
Recent studies have proposed that diurnal rhythm promotes differential modulation in activity, thermogenesis and fat oxidation in BAT. It was observed that plasmatic lipid metabolism was improved during daytime with a higher expression of lipoprotein lipase, FA uptake, and modulates lipid plasmatic concentration in BAT [ 203 ]. In the same line, Matsushita and colleagues, assessed forty-four healthy men who received diet-induced thermogenesis (DIT) under room temperature (27 °C) and cold (19 °C) in the morning and in the evening by using 18 F-fluoro-2-deoxy-D-glucose positron emission tomography. It was observed that thermogenic parameters presented better performance during the morning [ 204 ].
Moreover, several studies have established that melatonin directly impacts BAT morphology and function, also, in a mechanism dependent on adrenergic activation mediated by NE release. Melatonin is related to an increase of BAT volume, and thermogenic capacity, associated with the increase of UCP1 mRNA expression and mitochondrial mass and functionality, as well as seric lipid concentration. These profiles are significatively impaired under melatonin deficiency but reverse with oral melatonin replacement [ 205 , 206 , 207 ]. Growing evidence confirms the intimate relationship between circadian rhythm and AT, with emphasis on metabolic homeostasis and modulation of BAT activity. The characterization of how this process happens emerges as a strong diagnostic tool as well as a therapeutic approach concerning sleep disorders and metabolic diseases.
Food-intake and browning
Several studies suggest that food items can affect AT function. Among them curcumin, present in saffron, proved to be involved in the browning process, as mice treated with 50 or 100 mg/kg/day of this compound increased inguinal WAT expression of several browning-associated genes, such as Ucp1, Pgc1a, Prdm16, Dio2, PPARα, and CIDEA, and displayed mitochondrial biogenesis in this tissue. Curcumin stimulation was unable to induce the same effects in the epididymal WAT, though. This process was mediated by the NE-β3-AR pathway since the levels of NE and β3-AR were elevated in the inguinal WAT [ 208 ]. Although studies are scarce regarding the impact of thyme in the WAT browning process, it was observed that 20 µM of thymol, a substance present in the essential oils of thyme, in the complete medium when placed in contact with 3T3-LI preadipocytes for 6–8 days was able to induce an increased gene and protein expression of the PGC-1α, PPARγ, and UCP1. Such increases were related to the activation of β3-AR, AMPK, PKA, and Mitogen-activated protein kinase (p38 MAPK) being accompanied by an increase in mitochondrial biogenesis [ 209 ].
Cinnamon oil contains trans-cinnamic acid, which exposure to 3T3-L1 white adipocytes at 100 µM high gene expression of Lhx8, Ppargc1, Prdm16, Ucp1, and Zic1 and markers of UCP1, PRDM16, and PGC-1α, indicating WAT browning [ 210 ]. Quercetin, a flavonoid present in the onion, also proved to be efficient in the browning process since mice fed for 8 days with 0.5% onion peel extract (OPE) during a high-fat diet (HFD) exhibited increased expression levels OF UCP-1, PRMD16, AND PGC1-α in retroperitoneal white adipose tissue (rWAT) [ 211 ]. Just as the combination of quercetin and resveratrol also induces the WAT browning phenotype [ 212 ]. The resveratrol, present in the bark of grapes and other plants, also increases the expression of UCP-1, PRDM16, and PPARγ, suggesting that resveratrol induces the formation of beige adipocytes through the phosphorylation of AMPK, once treatment coupled with inhibition or the deletion of AMPK did not produce the same effects [ 208 ]. The same was observed in the substances found in the mushroom and honey, which induced increased expression of brown fat markers via AMPK and PGC-1α [ 213 ].
The peppers have capsaicin, an active compound responsible for the burning sensation that is also involved in the browning of WAT. One study used wild-type (WT) and TRPV1 −/− mice fed with HFD containing 0.01% capsaicin. The WT animals showed an increase in the expression of Ucp-1, Pgc-1α, Sirt-1, Prdm16, and exhibited browning of WAT via activation of the transient receptor potential vanilloid 1(TRPV1), which is related to the synthesis of catecholamine or sirtuin 1 (SIRT1)-mediated deacetylation of PPARγ, facilitating PPARγ-PRDM-16 interaction. The same did not happen in animals TRPV1 −/− , demonstrating that capsaicin depends on the role of TRPV1 in the browning process [ 214 ]. Menthol, an organic compound extracted from Mentha piperite oil, was shown to activate Transient Receptor Potential Cation Channel Subfamily M Member 8 (TRPM8), and this matched the increase in the thermogenic Ucp1 gene and the expression of Pgc-1α through PKA phosphorylation induced by free intracellular Ca 2+ in adipocytes treated with menthol for 8 h [ 215 ]. Other substances, such as carotenoids, are involved in the WAT browning process. Fucoxanthin, β-carotene, and citrus fruits are efficient in modulating the Ucp1 expression ( 216 , 217 , 218 ).
Another food component that is involved in the browning process of WAT is berberine, a molecule derived from the plants Coptis chinensis and Hydrastis canadensis . Obese male C57BLKS/J-Leprdb/Leprdb mice (db/db) were injected for 4 weeks with berberine (5 mg/kg/day). The group discovered berberine promotes BAT thermogenesis and WAT browning, since the igWAT, but not the epididymal, showed high levels of mRNA and UCP1 protein expression and increased mitochondrial biogenesis after injections. The brown adipocyte markers PGC-1α, CIDEA, Cox8b, and lsdp5 were also elevated and AMPK and PGC-1α are involved [ 219 ]. In another study, the polyphenols from tea extracts (0.5%) present in the high-fat diet for 8 weeks reduced the size of adipocytes and induced browning markers in WAT, and the size of lipid droplets and whitening markers were reduced in the BAT [ 220 ]. Another analysis with the extract induced 77.5 or 155 mg/kg/day for 8 days, in which there was an increase in UCP-1 and PPARγ [ 221 ].
Genistein, present in soy, indirectly induces browning since it is capable of increasing irisin levels, through PGC-1α / FNDC5, which increases Ucp1 and Tmem26 expression ( 222 ). In Magnolia Officinalis, two magnolol compounds (20 µM) and Honokiol (1–20 µM) when used to stimulate 3T3-L1 adipocytes increased protein levels of PGC-1α, PRDM16, and UCP-1 [ 223 ]. Honokiol also increased protein expression levels of CIDEA, COX8, FGF21, PGC-1α, and UCP1 [ 224 ]. The herb panax ginseng contains ginsenoside Rg1 (10 μM of ginsenoside Rb1), which is capable of considerably increasing the mRNA expression of UCP1, PGC-1α, and PRDM16 in mature 3T3-L1 adipocytes via PPARγ [ 225 ], as well as activating the AMP-activated protein kinase pathway [ 226 ].
The fish oil is rich in n-3 polyunsaturated fatty acids (PUFAs), components that are associated with the formation of beige adipocytes, among them is eicosapentaenoic acid (EPA). Mice fed different diets, including with EPA, for 8 weeks showed increased expression of β3-AR, PGC-1α, and UCP1 and exhibited high expression of PPAR [ 227 ], though this effect is controversial since another animal study investigating a diet containing pure EPA (3.6% as EPA ethyl ester) did not show the expression of beige adipocyte marker genes of inguinal and visceral WAT, but only in BAT [ 228 ]. Docosahexaenoic acid (DHA) (1.2%) together with EPA (2.4%) increased oxygen consumption and rectal temperature, as well as UCP1 and β3AR levels via the central nervous system. However, knockout mice for TRPV1 did not achieve the same effect, showing that such events were mediated by SNS, TRPV1, and catecholamines [ 229 ]. Conjugated linoleic acids (CLAs) also showed potential to induce browning process in the WAT [ 230 ].
Once the overwhelming impact of infectious diseases has been alleviated by the development of efficient therapeutics, life expectancy has been continuously increasing (World Health Organization, 2019). Age-associated diseases, including type 2 diabetes (T2D), cardiovascular diseases (CVDs), neurodegenerative pathologies, and obesity statistics are alarming and correlates with changes in the lifestyle of individuals throughout the world, including the diet, and impair the health spam rise. Western diets (WDs) are composed by food items enriched in processed sugar, white flour and salt and poor in fibers, vitamins and minerals [ 231 ]. At the same time, the diet may be the remedy against the burden caused by these chronic diseases. While overnutrition often correlates with inflammatory and metabolic detrimental effects at molecular level, undernutrition without starvation presents many benefits. Calorie restriction (CR) and intermittent fasting (IF) are promising interventions against the overweight and obesity numbers, climbing specially in Western countries [ 232 ].
CR, defined as reduced calorie consumption without malnutrition, is the best studied dietary intervention that increase health spam in experimental models. A plethora of human studies place CR as beneficial for expanding the health spam [ 232 ]. These studies proceeded Weindruch and Sohal positive correlations between CR and health spam [ 233 ] Click or tap here to enter text.. AT plasticity is one of the connections between CR and health benefits. Fabbiano and colleagues analyzed mice under CR and described that this regimen induces functional beige fat development in WAT, phenomenon that occur via enhanced type 2 immune response and SIRT1 expression in AT macrophages [ 234 ].
The stress resistance provided by the IF practice places this regimen as a feasible dietary intervention against various devastating complex pathologies. Differently from CR, intermittent fasting (IF) does not influence the meal size, but decrease the number of meals in a given period [ 232 ]. The fasting state leads to a metabolic switch, which increases the usage of free fatty acid (FFA) as energy source in comparison to glucose. In addition, IF favors the synthesis of ketone bodies (KBs) by the liver, molecules that act as an energy source during nutrient deprivation and induce a plethora of beneficial effects on the organism by acting upon the muscle, liver, heart, brain, intestine and AT [ 235 , 236 , 237 ]. Moreover, during prolonged fasting periods, the levels of the bioenergetic sensors NADH, ATP, and acetyl-CoA decrease and the amounts of NAD+, AMP, CoA rise, molecules that act as epigenetic cofactors and lead to the activation of stress resistance mediators, as sirtuins (SIRTs), NRF2 (nuclear factor erythroid 2–related factor 2), and AMPK (AMP-activated protein kinase). IF also impacts positively on AT remodeling. A DIO animal model submitted to repetitive fasting cycles displayed increased glucose tolerance, and diminished adipocyte hypertrophy and tissue inflammation [ 238 ]. Mouse studies show that IF induces WAT mass decrease, elevation of AT UCP1 expression and thermogenic capacity [ 239 , 240 ], and augmented beige pre-adipocytes recruitment to WAT [ 241 , 242 , 243 ] (Fig. 2 ).
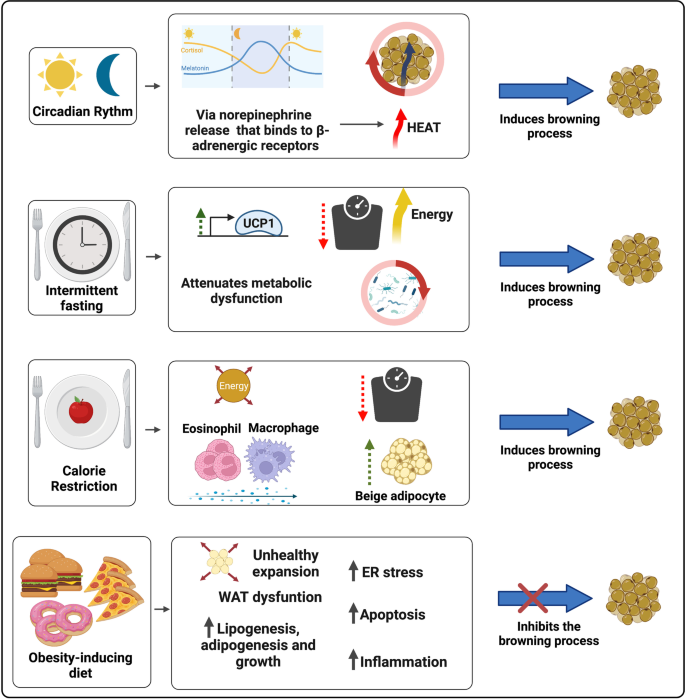
The impact of circadian rhythm and different diets on the WAT browning modulation. The secretion of melatonin, a circadian rhythm regulating neurohormone, is mediated by the release of Norepinephrine (NE), which binds to β-adrenergic receptors. Adrenergic activation is one of the main mechanisms of WAT browning induction and BAT activation. Intermittent fasting (IF) associates with weight reduction, improved metabolic status due to increased glycemic tolerance, decreased white adipocyte hypertrophy and AT inflammation, and augmented expression of thermogenic genes (such as UCP1) and recruitment of beige adipocytes. IF is also modulates the intestinal microbiome composition and diversity, a shift closely related to the induction of browning in the WAT. Caloric restriction (CR) is also associated with weight loss, promotes greater recruitment of beige adipocytes through the participation of M2 macrophage and eosinophil infiltration and in WAT. Finally, obesity-inducing diets correlate with increased lipid accumulation, WAT unhealthy expansion and dysregulation. Abnormal expansion of WAT promotes ER stress, greater induction of adipose cell apoptosis and inflammation through NF-κB transcription factor activation and increased pro-inflammatory cytokines secretion
An elegant study conducted by Li and colleagues informed that mice under IF cycles display an intestinal microbiome composition shift associated with increased levels of the fermentation products lactate and acetate. They also show that the modulation of the gut microbiota by IF is crucial for its browning effect, as microbiota-depleted mice present impaired IF-induced AT beiging and fecal microbiota transfer from these mice to antibiotics treated animals display increased browning of WAT [ 244 ] (Fig. 2 ). Unexpectedly, a human study conducted by von Schwartzenberg and colleagues showed that CR may diminish bacterial abundance, deeply change gut microbiome composition and diversity, impair nutrient absorption, and favor the outgrowth of the pathobiont ( Clostridioides difficile ). This diet also led to a decrease in bile acid (BA) levels [ 245 ].
BA, nonesterified fatty acids, are synthesized during the browning of WAT, a phenomena associated with the potentiation of the lipolytic machinery [ 246 ]. These fatty acids can not only activate UCP-1 allosterically, but also serve as fuel for oxidative phosphorylation and consequently heat generation in BAT [ 1 ]. Furthermore, in the liver they are used for the generation of acylcarnitines and VLDL which is used as source for thermogenesis [ 247 ]. Moreover, studies show that the increase in brite and brown adipocytes in WAT leads to an elevation in lipoprotein lipase (LPL) activity and subsequently an increase in circulating lipids available for BAT through intravascular hydrolysis of chylomicron triglycerides [ 248 ]. Consequently, these mechanisms result in the generation of cholesterol-enriched lipoprotein remnants, which upon activation of BAT accelerates the flow of cholesterol to the liver [ 249 ].
BA are steroid acids derived from dietary cholesterol catabolism. These acids are synthesized in the liver and act to aid digestion and absorption of fat in the intestine, in addition to playing an essential role in lipid metabolism. BA act in other tissues, such as AT, as signaling molecules through interaction with the nuclear Farnesoid X receptor (FXR) and the G protein-coupled membrane receptor (TGR5) [ 250 ]. Recent studies have shown that BA play a relevant role in BAT activation and increased thermogenesis in adipocytes. In rodents, the activation of BAT by BA is dependent on its interaction with the TGR5 receptor and expression of the enzyme type 2 iodothyronine deiodinase (DIO2). Additionally, experiments with oral supplementation of BA in humans indicated increased BAT activity in humans [ 251 , 252 ]. Another experiment performed under thermoneutrality, demonstrated an improvement in glycemic metabolism and lipogenesis in the liver and fat accumulation in the TA and also induced an improvement in thermogenic parameters and mitigation of the impact of diet-induced obesity after feeding mice with HFD associated with BA [ 253 ].
Moreover, BAT activation also promotes liver protection. In a study performed with animals under alcohol-induced hepatic steatosis or liver injury, activation of the TGR5 receptor induced improvement of clinical condition. The increase in thermogenesis in BAT promotes an increase in lipid metabolism with lower availability of circulating FFA and, consequently, lower absorption of these molecules by the liver [ 254 ]. However, if on the one hand BAs have been shown to be effective in inducing browning, on the other hand the excess of these acids is capable of promoting an antagonistic effect, such as mitochondrial dysfunction and expression of genes associated with cellular senescence in adipose cells [ 255 ].
Transcriptional regulation of WAT browning
ATs are embryologically distinct from other tissues and are formed according to specific stimuli during embryo development, including Bone morphogenetic proteins (BMPs), pleiotropic molecules that interact with type I and type II BMP receptors and influence embryogenesis [ 256 , 257 ]. BMPs interact with type I and type II BMP receptors serine/threonine kinase activity and influence lineage determination [ 258 ]. Tang and colleagues showed that transfection of C3H10T1/2 cells with BMPs coped with phenotypes: while BMP2 associated with the osteogenic lineage, BMP4 led to adipogenic differentiation [ 259 ].Noteworthy, BMP4 overexpression was found to increase UCP1 and other beiging markers, as Hoxc9, Tbx1, and Tbx15 [ 260 ]. These different phenotypes induced by the BMPs, including the induced beige adipocytes, highlight how relevant transcriptional regulation is for determining the cells functions and characteristics. The main proteins that regulate gene expression are the transcriptional factors (TFs), DNA binding proteins that modulate gene transcription by interacting with the gene promoter or cis-regulatory elements, such as enhancers and silencers, and include PPAR proteins, PGC-1α, and PRDM16 [ 261 , 262 ].
In addition to its roles in ATs development [ 263 ], PPARγ is a central TF for adipogenesis and lipid storage regulation, influences cell thermogenic capacity, and impacts lipid metabolism and insulin sensitivity [ 264 ]. This TF is expressed in elevated levels in ATs [ 265 ], and upon ligand binding PPARγ recruits different cofactor sets for controlling the expression of specific genes. PPARγ cooperates with the basic leucine-zipper factor C/EBPα and interacts with the majority of adipocyte-selective genes [ 266 , 267 ]. The use of PPARγ full agonists is associated with improved insulin sensitivity and induces WAT browning, but can cause detrimental effects, such as undesirable weight gain and augmented visceral adiposity [ 264 ].
Although PPARγ is sufficient for converting white adipocytes into cells that display a brown-like phenotype in vitro [ 268 ], PPAR-α and PPAR-β/δ also influence the browning process. PPAR-β/δ agonist enhances beta-oxidation and improves glucose tolerance, key characteristics of the white-to-beige plasticity [ 269 ]. PPARα acts synergistically with PPARγ in inducing robust WAT browning in vivo [ 268 ], and is currently considered a prominent target for treating metabolic disorders [ 269 ].
A way that PPAR agonists provoke WAT browning is by stabilizing PRDM16 [ 270 ], a protein that activates a complete set of thermogenic genes in WAT [ 271 ]. PRDM16 is essential for browning particularly in scWAT, once its induction in visceral depots does not correlate with thermogenesis [ 272 ]. Mice lacking Prdm16 in scWAT are unable to induce browning within subcutaneous depots after stimuli [ 273 ]. Ectopic PRDM16 expression induce thermogenic genes in several cell types [ 274 ]. PRDM16 AT overexpression in rodents copes with augmented energy expenditure and DIO resistance [ 272 ] PRDM16 acts by binding to specific regulatory sequences in DNA and by interacting with other proteins [ 271 ], such as PGC-1α [ 275 ].
PGC-1α plays a key role in the adapting thermogenesis. First described in cold-induced adaptive thermogenesis analyses [ 276 ], this transcriptional coactivator participates in the regulation of a plethora of cellular functions, including mitochondrial biogenesis, oxidative phosphorylation, and gluconeogenesis [ 277 , 278 ]. Once PGC-1α influences genes related to energy metabolism, it is expressed mostly in tissues that require an elevated amount of energy, like AT, liver, skeletal muscle, and brain [ 279 ]. Pgc1α is activated by the action of the cAMP-PKA-p38/MAPK signaling pathway and physically interacts with Nuclear Respiratory Factors 1 and 2 (NRF1 and NRF2) and co-activates PPARγ, PPARα, and ERRα/β/γ [ 280 ]. When overexpressed, Pgc1α induces mitochondrial biogenesis [ 281 ].
Another key regulator of the browning process is CIDEA. Initially described as a mitochondrial protein, CIDEA was further discovered to be associated with cell lipid droplets (LD) [ 282 , 283 , 284 ]. This molecule leads to the occurrence of browning by inhibiting the suppression of UCP1 gene expression mediated by liver-X receptors (LXRs) and increasing PPARγ binding strength to the UCP1 enhancer [ 285 ]. As detailed in this review, UCP1 is found in the inner mitochondrial membrane and acts by uncoupling the electron transport chain and oxidative phosphorylation, releasing energy as heat [ 1 ]. The existence of molecular markers for the browning process can be useful for investigating AT plasticity status and correlate with health and disease.
Zfp516 is a TF that directly binds to the UCP1 and PGC1α promoters and induce WAT browning upon cold exposure. Zfp516 overexpression copes with augmented multilocular lipid droplets (LDs) biogenesis and increased oxygen consumption and UCP1 levels [ 286 ]. HSF1 was described to cooperate with PGC1α in igWAT, favoring the induction of the thermogenic and mitochondrial gene programs, which leads to augmented energy consumption. HSF1-deficient mice are cold intolerant due to decreased β oxidation and UCP1 expression. HSF1 activation associates with scWAT browning, non-shivering thermogenesis and energy consumption [ 287 , 288 ]. IRF4 also cooperates with PGC1α and was described to inhibit lipogenesis in adipocytes [ 289 ]. While IRF4 overexpression favors beiging in epididymal WAT, the absence of this factor is linked to diminished energy expenditure and increased risk to hypothermia [ 290 ]. The TF NFIA presents a crucial role in the initial steps of thermogenic gene regulation, as its increased levels in thermogenic adipocytes precedes PPARγ upregulation in these cells [ 291 ].
Members of “Early B-Cell Factor” (EBF) protein family play key roles in the regulation of thermogenesis. The TF EBF2 uncouples adipocyte mitochondrial respiration and is sufficient for WAT browning. Increased EBF2 levels in WAT leads to activation of the thermogenic program, favoring increased oxygen consumption and resistance for weight gain. EBF2-KO mice show impaired WAT browning and ablates the brown fat-specific characteristics of BAT [ 292 , 293 , 294 ]. EBF2 activity is regulated by the action of ZFP423, which binds to EBF2 and recruits NuRD (nucleosome remodeling deacetylase) corepressor complex to suppress EBF2 activity in thermogenic genes regulatory sequences [ 295 ]. Absence of ZFP423 associates with PPARγ binding to thermogenic gene enhancers, WAT browning and non-shivering thermogenesis [ 296 ]. The protein transducin-like enhancer of split 3 (TLE3) was first described by Villanueva and colleagues to increase PPARγ adipogenic activity [ 297 ]. Deletion of TLE3 copes with increased energy expenditure and mitochondrial oxidative metabolism in adipocytes, characteristics associated with the browning process [ 298 ] KLF11, TAF7L, ZBTB16, EWS, PLAC8, ERRα, ΕRRγ and other TFs that were described to promote WAT browning. In contrast, FOXO1, TWIST1, p107, LXRα, pRB, RIP140, REVERBα acting repressing AT beiging by impacting on the activity of EBF2, PRDM16, PGC1α and other activating TF [ 299 , 300 , 301 ].
In order the transcription to occur, chromatin needs to be accessible to polymerases and TFs. Remodeling enzymes, which are classified in covalent histone modifiers and ATP-dependent chromatin remodeling complexes, actively modify chromatin status in response to environmental cues [ 302 ]. Histone covalent modifiers are enzymes that chemically modify positively-charged amino acids (mainly lysine) present in these proteins. Histone acetyltransferases (HATs) and histone deacetylases (HDACs) catalyze the removal or insertion of acetyl group, respectively, influencing histone acetylation pattern [ 303 ]. Acetylation decreases histones’ positive charge, leading to histone-DNA looser interaction, chromatin decompaction and gene activation. These epigenetic elements classified into three major families based on the acetyl group transfer mechanisms: the CREB binding protein (CBP)/P300 family (CBP, P300), the GCN5-related N-acetyltransferases (GNAT) family (GCN5, PCAF, and Hat1), and the MYST family (MYST1, MYST2, TIP60) [ 304 ]. The HAT CBP was shown to inhibit the browning process, once it is key in white adipocyte differentiation [ 305 ]. Histone acetylation pattern has been described to present a critical role in adipocyte identity, as cold exposure leads beige adipocytes to show histone acetylation pattern associated with brown phenotype [ 306 ]. The HATs GCN5/PCAF were also shown to participate in the browning process [ 307 ].
HDACs catalyze the deacetylation of amino acid residues in histones, reactions favor gene repression. These enzymes are categorized into four groups, namely class I (HDAC1–3 and 8), class IIa (HDAC4, 5, 7, and 9), class IIb (HDAC6 and 10), class III (SIRT1–7), and class IV (HDAC11) [ 304 ]. HDAC1 expression is augmented in WAT and copes with decreased levels of proteins associated with non-shivering thermogenesis, as UCP1 and PPARγ [ 308 ]. HDAC3 levels also suppress WAT browning, as absence in WAT correlates with H3K27ac on enhancers of Ucp1 and Ppar g , signature associated with tissue improved oxidative capacity, mitochondrial biogenesis, and thermogenesis [ 309 ]. Experiments involving the deletion of HDAC9 shows that this enzyme also contributes for the metabolic dysfunction characteristic of HFD-fed rodents [ 310 ]. HDAC11 is another element that impairs WAT beiging, once its removal favors at thermogenesis in diet-induced obese (DIO) mice [ 311 ]. Other key HDAC is the SIRT family (SIRT1, SIRT2, SIRT3, SIRT5, and SIRT6). SIRT1 deletion in HFD-treated mice is associated with diminished amounts of PGC-1α, FGF21, and UCP1 in epididymal WAT (eWAT) [ 312 ].
Histones can also be post-translationally modified by histone methyltransferases (HMTs) and histone demethylases, which influence gene activation or suppression depending on the modified residue position and valency [ 313 ]. There are two categories of HMTs: lysine methyltransferases (KMTs) and arginine methyltransferases (RMTs). Rodent studies show that inactivation of the KMT MLL3 favors improved insulin sensitivity and augmented energy expenditure [ 314 ]. Absence of another KMT, the EHMT1, was shown to impair the thermogenic program in WAT [ 315 ]. Histone demethylases (HDM) catalyze histone demethylation processes and are also classified according to the characteristics of the modified amino acid. The KDM LSD1 expression, which was found to be induced by chronic cold exposure and β3-adrenergic stimulation, leads to increased mitochondrial activity in WAT by cooperating with NRF1 [ 316 , 317 , 318 ]. In addition, rodents presenting increased LSD1 levels are associated with igWAT browning in lean animals and with lower weight gain in the context of DIO [ 317 ] Also induced by β3-adrenergic stimulation, the KDM3A JMJD1A directly regulates ppar a and ucp1 genes and was found to be crucial for WAT browning [ 319 ]. Specific DNA sequences called CpG islands can also be methylated for gene transcription regulation by DNA methyltransferases (DNMTs), which switches off gene expression. Ten-eleven translocation (TET) family enzymes switch on the gene expression by demethylation [ 320 ].
Gene transcription can also be impacted by the action of ATP-dependent chromatin remodeling complexes, which, differently from the covalent histone modifiers, alter the interaction between the DNA and the nucleosome non-covalently using ATP hydrolysis as an energy source. The chromatin remodelers are categorized in INO80, CHD, ISWI, and SWI/SNF [ 321 ]. The mammalian SWI/SNF (mSWI/SNF or BAF) complex can act with either Brahma homolog (BRM) or BRM-related gene 1 (BRG1) ATPases [ 322 ]. Abe and colleagues suggested that BRG1 is necessary for thermogenesis induction [ 319 ].
Other key actors in gene transcription regulation are the microRNAs (MiR), small non-coding regulatory ribonucleic acids (maximum 200 nucleotides) that influence gene expression post transcriptionally. These elements regulate gene expression by several mechanisms, including binding to mRNA strands to repress protein translation and to favor decapping, deadenylation and ultimately degradation of target mRNA in P-bodies, and direct translation inhibition. However, many studies suggest that miRNAs are also capable of activating transcription protein translation, fact that highlights miRNAs as central participants in the fine regulation of gene expression in response to specific stimuli [ 323 ]. Raymond and others described that miR-32 inhibition copes with impaired WAT browning [ 324 ]. MiR-181, which is induced by tryptophan‐derived metabolites produced by the intestinal microbiota, was also connected with WAT physiology, once it promotes energy expenditure and insulin sensitivity in rodent models and its removal may cope with the development of obesity in these animals [ 325 ]. Another miRNA that influences WAT homeostasis is miR-26, which deletion leads to WAT enlargement and overexpression inhibits the progression of obesity in the DIO in mice [ 326 ]. miR-196a, miR-455, miR-30b/c are other examples of miRNAs that are induced by browning inducers and promote this WAT plasticity process [ 327 , 328 ].
In contrast, miR-27, miR-133, and miR-150 inhibit TFs associated with the browning process [ 61 , 63 , 329 ]. Fu and colleagues showed that miR-34a inhibits WAT beiging via FGF21 [ 330 ], miRNA-155, miRNA Let-7i-5p and miR-125b- 5p are molecules that impair WAT browning process, which inhibition promotes beiging [ 331 , 332 , 333 ] (Fig. 3 ). Other small non-coding RNAs (sncRNAs) impact on gene expression and are extensively reviewed elsewhere.
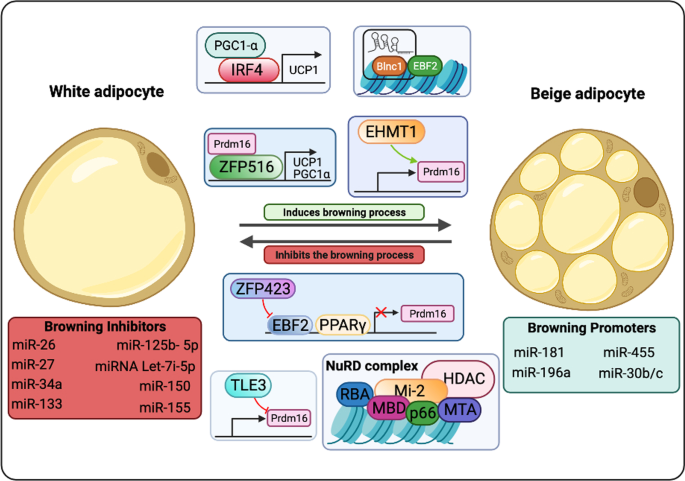
WAT browning transcriptional regulation. The transcriptional regulation of the WAT beiging process involves the action of a plethora of specific proteins and nucleic acids. This orchestra of trans-acting factors modulates genes associated with oxidative capacity, mitochondrial biogenesis and non-shivering thermogenesis. While the action of some factors, such as PGC-1α, IRF4, PRDM16, ZFP516, EHMT1 and RNAs, copes with WAT browning, TLE3, ZFP423, and the NuRD complex, another set of RNAs e others inhibit this process, favoring adipogenesis and white adipocyte differentiation
Non-coding RNAs that present average length longer than 200 nucleotides are called LncRNAs [ 302 , 334 ] These nucleic acids have been described to influence gene transcription through several mechanisms, including the induction of more efficient protein translation by binding to an internal ribosome entry site (IRES), increase of mRNAs stability, polyubiquitination process inhibition thus increasing protein stability, binding to specific proteins in the cytoplasm, and acting as a sponge sequestering miRNAs [ 335 , 336 , 337 , 338 , 339 ] . Zhao and collaborators identified lncRNA 1 (Blnc1) as a driver for beige adipocyte differentiation and WAT browning [ 293 ].
All the regulatory elements discussed here cooperate for generating a phenotype in response to environmental stimuli, such as physical exercising, intermittent fasting, calorie restriction, thyroid hormones, microbiota-associated metabolites, thyroid hormones and others [ 299 ] The lncRNA Blnc1 forms a Blnc1/hnRNP-U/EBF2 ribonucleoprotein complex and is required for EBF2 transcriptional activity [ 292 , 340 ]. The TF ZBTB7B also interacts with this lncRNA through the hnRNP-U/Blnc1 for beige adipocyte differentiation and thermogenesis [ 341 ]. LncRNAs also interact with other TFs, such as PGC-1α, ZFP516, and PRDM16 for full transcription [ 274 , 280 , 286 ]. PRDM16, a coregulator of PPAR, cooperates with EHMT1 for WAT browning induction. As EHMT1, other covalent histone modifiers form complexes with ATP-dependent chromatin-remodelers [ 342 ]. The chromatin-remodelers BRG1 and the BAF also interact with the TF EBF2 for gene transcription [ 292 , 293 ]. PGC1α favors increased transcription by recruiting HATs, as CBP/p300 and GCN5 [ 343 ].
Noteworthy, AT functional and morphological plasticity is represented by the extreme dynamic beige adipocyte chromatin state. Beige adipocytes show a chromatin signature similar to the pattern presented by brown adipocytes after cold exposure and display a chromatin signature associated with white adipocytes after re-warming to 30 °C. However, if the beige adipocytes were cold-induced, these cells displayed epigenetic marks that favored rapid thermogenic genes expression upon β-adrenergic stimulation, even after temperature rise, suggesting the occurrence of epigenomic memory [ 306 , 344 ].
WAT browning, health impact and applications
For the past decades, the browning process has been deeply explored for managing both metabolic syndromes, and hypermetabolic diseases. Proposing treatment, the cold was the pioneer mechanism associated with a great elicitation of this event in mice. However, the applicability and outcomes of this approach in humans are far from exciting. Technological advances provide efficient mechanisms that can be employed to improve a biological system. Recent studies applied bioengineers to achieve the improvement of browning in adipose tissue. The injection of M2 AT macrophages (ATMs) from transgenic mwRIP140KD mice, a specific knockdown of RIP140 that is related to activation of M1 polarization, in HFD-fed obese wild type mice could recover the disruption induced by obesity through browning induction [ 345 ]. WAT-derived stem cells (ASCs) from humans and rats could be differentiated into BAT under browning conditions in three-dimensional (3D) polyethylene glycol (PEG) hydrogel [ 346 ].
In addition to bioengineering, a field that has been explored is the transplantation or re-implantation of BAT, also termed ex-BAT, to increase this endogenous tissue. The harvested scWAT is differentiated into brite and then is re-implanted in the same area promoting the local increase of BAT, displaying great outcomes [ 347 ]. Aiming for a less invasive procedure, the use of a microneedle patch to address the browning agents directly to the scWAT for inducing browning of this tissue is another strategy investigated presently [ 348 ]. These strategies aim to promote the increase of endogenous BAT, as well as its activity with an applicable method, reducing the degree of invasiveness and risks of rejection by the body.
Several studies have targeted increasing BAT mass and activity, but when the aim is inhibiting the browning process to avoid a worsening prognosis, what is proposed? Expanding knowledge of the pathways involved is the main way of inhibiting that event. It is well established the role of parathyroid hormone (PTH) in the elicitation of browning. In the tumor context, it was identified as a peptide-derived tumor, parathyroid hormone-related protein (PTHrP) that favors browning and cachexia [ 16 ]. Blocking PTHR specifically in ATs inhibits browning and wasting of this tissue, as well preserves muscle integrity, and ameliorates muscle-related strength, protecting mice from cachexia [ 349 ].
Currently, some pharmacological approaches have displayed alternative and prominent ways to restrain browning. Metformin demonstrated to be efficient to prevent the browning of the scWAT, as well as the inhibition of mevalonate pathways by the use of Statin or Fluvastatin, which implicates in the disruption of browning [ 350 , 351 ]. However, further studies should be conducted to promote a broader and more detailed debate on the topic.
Given the data presented, it is evident that the benefits and dangers associated with activation or inhibition of the browning process are closely linked to the type of disorders displayed in the individual's body at the metabolic, cellular, or physiological level. Taking as criteria patients with metabolic syndromes and obesity, browning process emerges as a promising therapeutic approach, mainly due to its several benefits, such as improvement of clinical conditions associated with lower side effects, and the multi-stimulatory character, which allows numerous safe and feasible ways of induction. In contrast, the ways and means of inhibiting browning to prevent the development of comorbidities in cases of chronic or acute hypermetabolism are still poorly explored. In this way, browning process needs to be deeply explored and can be used as a key factor in different therapeutical approaches in health and diseases. Emerging insights into the metabolic and immunological role of browning of the white adipose tissue are also discussed, along with the developments that can be expected from these promising targets for therapy of metabolic and chronic disease in the forthcoming future.
Mouse and human AT research
A major discussion pointed by the scientific community about the investigation of AT in mice is how much the results obtained in the animal model would reproduce the anatomorphophysiologies of these tissues in humans, since, unlike humans, mice have a significant amount of BAT during embryonic and adult, as well as they differ in several other factors, such as expression of molecular markers, activation profile and location. Human BAT was discovered to be more similar to beige compared to classical BAT markers [ 352 , 353 ]. Another relevant point concerns the fact that the BAT deposits most used for research purposes are the BAT located in the interscapular region (iBAT), while in humans there is a greater abundance of this tissue in the clavicular and neck regions, presenting compositional differences that represent obstacles in the overlapping of scientific findings. In this sense, Mo and colleagues identified an analogous deposit of BAT in mouse embryos, which is maintained during adulthood, and in humans called supraclavicular BAT (scBAT). The scBAT shows similarities to scBAT in humans in terms of location, morphology and thermogenic capacity [ 354 ].
Another alternative developed in order to attenuate the anatomical, physiological and molecular differences between the organisms was to submit mice under conditions called “physiologically humanized” in which middle-aged animals under thermoneutrality (30˚C) and diets aligned with the dietary pattern of human beings are used. humans. In which the authors observed a greater similarity between several cellular and molecular parameters between the BAT of humans and mice [ 355 ]. The technique generated controversies that were discussed by Kajimura and Spiegelman and replicated by the authors of the original article ( 356 , 357 ).
Conclusions and perspectives
Current research place WAT browning as an extremely dynamic process that is influenced by several factors , including temperature, physical exercising, thyroid hormones, circardian rhythm, food components and dietary regimens. The participation of AT plasticity on the organism metabolic health and inflammatory status spot this process as a promising therapeutic target for decreasing the risk associated with many chronic diseases. Further efforts in investigating AT plasticity must alleviate the burden of these devastating life-style associated pathologies.
Availability of data and materials
Not applicable.
Abbreviations
5′-Deiodinase type 2
Amino acids
Adenylyl cyclase
AMP-activated protein kinase
Atrial natriuretic peptide
Adipose stromal cells
Adipose tissue
Activating transcription factor
Adipose tissue macrophages
Adenosine triphosphate
Brown adipose tissue
Blood–brain barrier
Bone morphogenetic proteins
Basal metabolic rate
Brain natriuretic peptide
BRM-related gene 1
Brahma homolog
CCAAT-enhancer-binding protein
Calcium ion
Cyclic adenosine monophosphate
CREB binding protein
Response element-binding protein
Cell death-inducing DNA fragmentation factor-like effector A
Conjugated linoleic acids
Central nervous systems
Carnitine palmitoyltransferase 1B
Calorie restriction
CAMP response element-binding protein
Cyclic AMP response element-binding protein H
Docosahexaenoic acid
Diet-induced obese
2 Iodothyronine deiodinase
Diet-induced thermogenesis
DNA methyltransferases
Epicardial AT
Early B-cell factor
Endocrine FGF
Eukaryotic initiation factor
Eicosapentaenoic acid
Epididymal WAT
Fatty acids
Free fatty acids
Fibroblast growth factor family
Fibroblast growth factor tyrosine kinase receptor
Fibronectin domain-containing protein 5
FGFR substrate 2α
Farnesoid X receptor
Non-derepressible 2
Glucose transporter
GCN5-related N-acetyltransferases
Histone acetyltransferase
Histone deacetylase
Histone demethylase
High-fat diet
Histone methytransferases
Interscapular BAT
Intermittent fasting
Interleukin
Internal ribosome entry site
Beta-Klotho
Lysine methyltransferases
Long-chain fatty acids
Lipid droplet
Long non-coding RNA
Lipopolysaccharide
Liver X receptor
Mitochondrial anion carrier family
Membrane proteins monocarboxylate transporter 8
Messenger RNA
Myogenic factor 5
Nonalcoholic fatty liver disease
Non-coding RNAs
Norepinephrine
Nuclear factor erythroid-related factor 2
Nuclear respiratory factor 1
Nuclear respiratory factor 2
Nucleosome remodeling deacetylase
Organic anion transporter family member 1C1 9
Onion peel extract
Mitogen-activated protein kinase
Pyruvate dehydrogenase
Polyethylene glycol
Peroxisome proliferator-activated receptor-gamma coactivator 1 α
Protein kinase A
Proopiomelanocortin
Peroxisome proliferator-activated receptor
PR domain containing 16
Parathyroid hormone
Parathyroid hormone receptor
Parathyroid hormone-related protein
Polyunsaturated fatty acids
Retinoic acid
Retinoic acid receptor
Receptor-interacting protein 140
Arginine methyltransferases
Retinoic acid receptor-related orphan receptor α
Retroperitoneal white adipose tissue
Retinoid X receptor
Supraclavicular BAT
Suprachiasmatic nucleus
Subcutaneous WAT
Small non-coding RNA
Sympathetic nervous system
Type 2 diabetes
Triiodothyronine
T-box transcription factor TBX1
Ten-eleven translocation
Transcription factor
Transforming growth fator beta
G protein-coupled membrane bile acid receptor
Thyroid Hormone
T help 2 cells
Transducin-like enhancer of split 3
Thyroid-hormone responsive elements
Transient receptor potential cation channel subfamily M member 8
Transient receptor potential vanilloid 1
Thyroid hormone nuclear receptors
Thyroid stimulating hormone
Thyroid stimulating hormone receptor
Uncoupling protein 1
Visceral WAT
- White adipose tissue
Zinc-α2-glycoprotein
β-3 Adrenergic receptor
Cannon B, Nedergaard J. Brown adipose tissue: function and physiological significance. Physiol Rev. 2004;84(1):277–359.
Article CAS PubMed Google Scholar
Moriya K, Arnold J, LeBlanc J. Shivering and nonshivering thermogenesis in exercised cold-deacclimated rats. Eur J Appl Physiol Occup Physiol. 1988;57(4):467–73.
Prunet-Marcassus B, Cousin B, Caton D, André M, Pénicaud L, Casteilla L. From heterogeneity to plasticity in adipose tissues: site-specific differences. Exp Cell Res. 2006;312(6):727–36.
Barbatelli G, Murano I, Madsen L, Hao Q, Jimenez M, Kristiansen K, et al. The emergence of cold-induced brown adipocytes in mouse white fat depots is determined predominantly by white to brown adipocyte transdifferentiation. Am J Physiol Metab. 2010;298(6):E1244–53.
CAS Google Scholar
Suárez-Zamorano N, Fabbiano S, Chevalier C, Stojanović O, Colin DJ, Stevanović A, et al. Microbiota depletion promotes browning of white adipose tissue and reduces obesity. Nat Med. 2015;21(12):1497–501.
Article PubMed PubMed Central CAS Google Scholar
Johann K, Cremer AL, Fischer AW, Heine M, Pensado ER, Resch J, et al. Thyroid-hormone-induced browning of white adipose tissue does not contribute to thermogenesis and glucose consumption. Cell Rep. 2019;27(11):3385-3400.e3.
Barberá MJ, Schlüter A, Pedraza N, Iglesias R, Villarroya F, Giralt M. Peroxisome proliferator-activated receptor α activates transcription of the brown fat uncoupling protein-1 gene: a link between regulation of the thermogenic and lipid oxidation pathways in the brown fat cell. J Biol Chem. 2001;276(2):1486–93.
Article PubMed Google Scholar
Schulz TJ, Huang TL, Tran TT, Zhang H, Townsend KL, Shadrach JL, et al. Identification of inducible brown adipocyte progenitors residing in skeletal muscle and white fat. Proc Natl Acad Sci U S A. 2011;108(1):143–8.
Villarroya F, Peyrou M, Giralt M. Transcriptional regulation of the uncoupling protein-1 gene. Biochimie. 2017;134:86–92.
Kalinovich AV, de Jong JMA, Cannon B, Nedergaard J. UCP1 in adipose tissues: two steps to full browning. Biochimie. 2017;134:127–37.
Yau WW, Singh BK, Lesmana R, Zhou J, Sinha RA, Wong KA, et al. Thyroid hormone (T 3) stimulates brown adipose tissue activation via mitochondrial biogenesis and MTOR-mediated mitophagy. Autophagy. 2019;15(1):131–50.
Pérez-Martí A, Garcia-Guasch M, Tresserra-Rimbau A, Carrilho-Do-Rosário A, Estruch R, Salas-Salvadó J, et al. A low-protein diet induces body weight loss and browning of subcutaneous white adipose tissue through enhanced expression of hepatic fibroblast growth factor 21 (FGF21). Mol Nutr Food Res. 2017;61(8):1–32.
Article CAS Google Scholar
Finlin BS, Memetimin H, Confides AL, Kasza I, Zhu B, Vekaria HJ, et al. Human adipose beiging in response to cold and mirabegron. JCI Insight. 2018;3(15):e121510.
Article PubMed Central Google Scholar
Otero-Díaz B, Rodríguez-Flores M, Sánchez-Muñoz V, Monraz-Preciado F, Ordoñez-Ortega S, Becerril-Elias V, et al. Exercise induces white adipose tissue browning across the weight spectrum in humans. Front Physiol. 2018;9:1781.
Article PubMed PubMed Central Google Scholar
Carrière A, Jeanson Y, Berger-Müller S, André M, Chenouard V, Arnaud E, et al. Browning of white adipose cells by intermediate metabolites: an adaptive mechanism to alleviate redox pressure. Diabetes. 2014;63(10):3253–65.
Article PubMed CAS Google Scholar
Kir S, White JP, Kleiner S, Kazak L, Cohen P, Baracos VE, et al. Tumour-derived PTH-related protein triggers adipose tissue browning and cancer cachexia. Nature. 2014;513(7516):100–4.
Article CAS PubMed PubMed Central Google Scholar
Abdullahi A, Samadi O, Auger C, Kanagalingam T, Boehning D, Bi S, et al. Browning of white adipose tissue after a burn injury promotes hepatic steatosis and dysfunction. Cell Death Dis. 2019;10(12):870.
Lucchini FC, Wueest S, Challa TD, Item F, Modica S, Borsigova M, et al. ASK1 inhibits browning of white adipose tissue in obesity. Nat Commun. 2020;11(1):1642.
Frontini A, Cinti S. Distribution and development of brown adipocytes in the murine and human adipose organ. Cell Metab. 2010;11(4):253–6.
Luo L, Liu M. Adipose tissue in control of metabolism. J Endocrinol. 2016;231:R77-99.
Kershaw EE, Flier JS. Adipose tissue as an endocrine organ. J Clin Endocrinol Metab. 2004;89(6):2548–56.
Andrade-Oliveira V, Câmara NOS, Moraes-Vieira PM. Adipokines as drug targets in diabetes and underlying disturbances. J Diabetes Res. 2015;2015:1.
Article Google Scholar
Jokinen R, Pirnes-karhu S, Pietiläinen KH, Pirinen E. Adipose tissue NAD+-homeostasis, sirtuins and poly(ADP-ribose) polymerases—important players in mitochondrial metabolism and metabolic health. Redox Biol. 2017;12(February):246–63.
Parimisetty A, Dorsemans AC, Awada R, Ravanan P, Diotel N, Lefebvre C. Secret talk between adipose tissue and central nervous system via secreted factors-an emerging frontier in the neurodegenerative research. J Neuroinflam. 2016;13:1.
Reilly SM, Saltiel AR. Adapting to obesity with adipose tissue inflammation. Nat Rev Endocrinol. 2017;13:633–43.
Park A. Distinction of white, beige and brown adipocytes derived from mesenchymal stem cells. World J Stem Cells. 2014;6(1):33.
Dalal S. Lipid metabolism in cancer cachexia. Ann Palliat Med. 2019;8(1):13–23.
Wu J, Boström P, Sparks LM, Ye L, Choi JH, Giang AH, et al. Beige adipocytes are a distinct type of thermogenic fat cell in mouse and human. Cell. 2012;150(2):366–76.
Cuevas-Ramos D, Mehta R, Aguilar-Salinas CA. Fibroblast growth factor 21 and browning of white adipose tissue. Front Physiol. 2019;10:37.
Bargut TCL, Souza-Mello V, Aguila MB, Mandarim-de-Lacerda CA. Browning of white adipose tissue: lessons from experimental models. Horm Mol Biol Clin Investig. 2017. https://doi.org/10.1515/hmbci-2016-0051 .
Shao M, Wang QA, Song A, Vishvanath L, Busbuso NC, Scherer PE, et al. Cellular origins of beige fat cells revisited. Diabetes. 2019;68(10):1874–85.
Vargas-Castillo A, Fuentes-Romero R, Rodriguez-Lopez LA, Torres N, Tovar AR. Understanding the biology of thermogenic fat: is browning a new approach to the treatment of obesity? Arch Med Res. 2017;48(5):401–13.
Long JZ, Svensson KJ, Tsai L, Zeng X, Roh HC, Kong X, et al. A smooth muscle-like origin for beige adipocytes. Cell Metab. 2014;19(5):810–20.
Shao M, Vishvanath L, Busbuso NC, Hepler C, Shan B, Sharma AX, et al. De novo adipocyte differentiation from Pdgfrβ+ preadipocytes protects against pathologic visceral adipose expansion in obesity. Nat Commun. 2018;9(1):1–16.
Oguri Y, Shinoda K, Kim H, Alba DL, Bolus WR, Wang Q, et al. CD81 controls beige fat progenitor cell growth and energy balance via FAK signaling. Cell. 2020;182(3):563-577.e20.
Tabuchi C, Sul HS. Signaling pathways regulating thermogenesis. Front Endocrinol (Lausanne). 2021;26(12):243.
Google Scholar
Himms-hagen J, Melnyk A, Zingaretti MC, Ceresi E, Barbatelli G, Cinti S. Multilocular fat cells in WAT of CL-316243-treated rats derive directly from white adipocytes. Am J Physiol Cell Physiol. 2000;279:C670.
Golozoubova V, Cannon B, Nedergaard J. UCP1 is essential for adaptive adrenergic nonshivering thermogenesis. Am J Physiol - Endocrinol Metab. 2006;291(2):350–7.
Schenk H, Franke H, Heim T. Structure and function of brown adipose tissue. Acta Histochem. 1977;59:195–208.
Klingenberg M, Echtay KS, Bienengraeber M, Winkler E, Huang SG. Structure-function relationship in UCP1. Int J Obes. 1999;23:S24–9.
Ricquier D, Bouillaud F. The uncoupling protein homologues: UCP1, UCP2, UCP3 StUCP and AtUCP. Biochem J. 2000;345(2):161–79.
Echtay KS. Mitochondrial uncoupling proteins—What is their physiological role? Free Radic Biol Med. 2007;43(10):1351–71.
Heaton JM. The distribution of brown adipose tissue in the human. J Anat. 1972;112(Pt 1):35–9.
CAS PubMed PubMed Central Google Scholar
Leitner BP, Huang S, Brychta RJ, Duckworth CJ, Baskin AS, McGehee S, et al. Mapping of human brown adipose tissue in lean and obese young men. Proc Natl Acad Sci USA. 2017;114(32):8649–54.
Klingenberg M, Echtay KS. Uncoupling proteins: the issues from a biochemist point of view. Biochim Biophys Acta Bioenerg. 2001;1504(1):128–43.
Chechi K, Grundberg E, Richard D, Chechi K, Vijay J, Voisine P, et al. UCP1 expression—associated gene signatures of human epicardial adipose tissue Find the latest version : UCP1 expression—associated gene signatures of human epicardial adipose tissue. JCI Insight. 2019;4(8):e123618.
Hoang T, Smith MD, Jelokhani-Niaraki M. Expression, folding, and proton transport activity of human uncoupling protein-1 (ucp1) in lipid membranes. J Biol Chem. 2013;288(51):36244–58.
Ježek P, Jabůrek M, Porter RK. Uncoupling mechanism and redox regulation of mitochondrial uncoupling protein 1 (UCP1). Biochim Biophys Acta Bioenerg. 2019;1860(3):259–69.
Macher G, Koehler M, Rupprecht A, Kreiter J, Hinterdorfer P, Pohl EE. Inhibition of mitochondrial UCP1 and UCP3 by purine nucleotides and phosphate. Biochim Biophys Acta Biomembr. 2018;1860(3):664–72.
Kozak UC, Kopecky J, Teisinger J, Enerbäck S, Boyer B, Kozak LP. An upstream enhancer regulating brown-fat-specific expression of the mitochondrial uncoupling protein gene. Mol Cell Biol. 1994;14(1):59–67.
Yubero P, Barbera MJ, Alvarez R, Vinas O, Mampel T, Iglesias R, et al. Dominant negative regulation by c-Jun of transcription of the uncoupling protein-1 gene through a proximal cAMP-regulatory element: a mechanism for repressing basal and norepinephrine-induced expression of the gene before brown adipocyte differentiation. Mol Endocrinol. 1998;12(7):1023–37.
Manchado C, Yubero P, Vinas O, Iglesias R, Villarroya F, Mampel T, et al. CCAAT/enhancer-binding proteins α and β in brown adipose tissue: Evidence for a tissue-specific pattern of expression during development. Biochem J. 1994;302(3):695–700.
Cassard-Doulcier AM, Gelly C, Bouillaud F, Ricquier D. A 211-bp enhancer of the rat uncoupling protein-1 (UCP-1) gene controls specific and regulated expression in brown adipose tissue. Biochem J. 1998;333(2):243–6.
Sears IB, MacGinnitie MA, Kovacs LG, Graves RA. Differentiation-dependent expression of the brown adipocyte uncoupling protein gene: regulation by peroxisome proliferator-activated receptor gamma. Mol Cell Biol. 1996;16(7):3410–9.
Wang H, Zhang Y, Yehuda-Shnaidman E, Medvedev AV, Kumar N, Daniel KW, et al. Liver X receptor α is a transcriptional repressor of the uncoupling protein 1 gene and the brown fat phenotype. Mol Cell Biol. 2008;28(7):2187–200.
Kiskinis E, Hallberg M, Christian M, Olofsson M, Dilworth SM, White R, et al. RIP140 directs histone and DNA methylation to silence Ucp1 expression in white adipocytes. EMBO J. 2007;26(23):4831–40.
Shore A, Karamitri A, Kemp P, Speakman JR, Lomax MA. Role of Ucp1 enhancer methylation and chromatin remodelling in the control of Ucp1 expression in murine adipose tissue. Diabetologia. 2010;53(6):1164–73.
Gebert LFR, MacRae IJ. Regulation of microRNA function in animals. Nat Rev Mol Cell Biol. 2019;20(1):21–37.
Oliverio M, Schmidt E, Mauer J, Baitzel C, Hansmeier N, Khani S, et al. Dicer1-miR-328-Bace1 signalling controls brown adipose tissue differentiation and function. Nat Cell Biol. 2016;18(3):328–36.
Zhang H, Guan M, Townsend KL, Huang TL, An D, Yan X, et al. Micro RNA -455 regulates brown adipogenesis via a novel HIF 1an- AMPK - PGC 1α signaling network. EMBO Rep. 2015;16(10):1378–93.
Chen SZ, Xu X, Ning LF, Jiang WY, Xing C, Tang QQ, et al. MiR-27 impairs the adipogenic lineage commitment via targeting lysyl oxidase. Obesity. 2015;23(12):2445–53.
Kang T, Lu W, Xu W, Anderson L, Bacanamwo M, Thompson W, et al. MicroRNA-27 (miR-27) targets prohibitin and impairs adipocyte differentiation and mitochondrial function in human adipose-derived stem cells. J Biol Chem. 2013;288(48):34394–402.
Trajkovski M, Ahmed K, Esau CC, Stoffel M. MyomiR-133 regulates brown fat differentiation through Prdm16. Nat Cell Biol. 2012;14(12):1330–5.
Enerbäck S, et al. Mice lacking mitochondrial uncoupling protein are cold-sensitive but not obese. Nature. 1997;387:90.
Liu X, Rossmeisl M, McClaine J, Kozak LP. Paradoxical resistance to diet-induced obesity in UCP1-deficient mice. J Clin Invest. 2003;111(3):399–407.
Hankir MK, Seyfried F. Do bariatric surgeries enhance brown/beige adipose tissue thermogenesis? Front Endocrinol (Lausanne). 2020;11(April):1–11.
Omran F, Christian M. Inflammatory signaling and brown fat activity. Front Endocrinol (Lausanne). 2020;11(March):1–16.
Reynés B, van Schothorst EM, Keijer J, Ceresi E, Oliver P, Palou A. Cold induced depot-specific browning in ferret aortic perivascular adipose tissue. Front Physiol. 2019;10(September):1–13.
Shankar K, Kumar D, Gupta S, Varshney S, Rajan S, Srivastava A, et al. Role of brown adipose tissue in modulating adipose tissue inflammation and insulin resistance in high-fat diet fed mice. Eur J Pharmacol. 2019;854(February):354–64.
van Marken Lichtenbelt WD, Vanhommerig JW, Smulders NM, Drossaerts JMAFL, Kemerink GJ, Bouvy ND, et al. Cold-activated brown adipose tissue in healthy men. N Engl J Med. 2009;360(15):1500–8.
Feldmann HM, Golozoubova V, Cannon B, Nedergaard J. UCP1 ablation induces obesity and abolishes diet-induced thermogenesis in mice exempt from thermal stress by living at thermoneutrality. Cell Metab. 2009;9(2):203–9.
Ohtomo T, Ino K, Miyashita R, Chigira M, Nakamura M, Someya K, et al. Chronic high-fat feeding impairs adaptive induction of mitochondrial fatty acid combustion-associated proteins in brown adipose tissue of mice. Biochem Biophys Rep. 2017;10:32–8.
PubMed PubMed Central Google Scholar
Winn NC, Vieira-Potter VJ, Gastecki ML, Welly RJ, Scroggins RJ, Zidon TM, et al. Loss of UCP1 exacerbates western diet-induced glycemic dysregulation independent of changes in body weight in female mice. Am J Physiol Regul Integr Comp Physiol. 2017;312(1):R74-84.
Bond LM, Burhans MS, Ntambi JM. Uncoupling protein-1 deficiency promotes brown adipose tissue inflammation and ER stress. PLoS ONE. 2018;13(11):1–11.
Bond LM, Ntambi JM. UCP1 deficiency increases adipose tissue monounsaturated fatty acid synthesis and trafficking to the liver. J Lipid Res. 2018;59(2):224–36.
Alcalá M, Calderon-Dominguez M, Bustos E, Ramos P, Casals N, Serra D, et al. Increased inflammation, oxidative stress and mitochondrial respiration in brown adipose tissue from obese mice. Sci Rep. 2017;7(1):1–12.
Tamucci KA, Namwanje M, Fan L, Qiang L. The dark side of browning. Protein Cell. 2018;9(2):152–63.
Lim J, Park HS, Kim J, Jang YJ, Kim J-H, Lee Y, et al. Depot-specific UCP1 expression in human white adipose tissue and its association with obesity-related markers. Int J Obes. 2020;44(3):697–706.
Han J, Meng Q, Shen L, Wu G. Interleukin-6 induces fat loss in cancer cachexia by promoting white adipose tissue lipolysis and browning. Lipids Health Dis. 2018;17(1):1–8.
Petruzzelli M, Schweiger M, Schreiber R, Campos-Olivas R, Tsoli M, Allen J, et al. A switch from white to brown fat increases energy expenditure in cancer-associated cachexia. Cell Metab. 2014;20(3):433–47.
Li L, Li B, Li M, Speakman JR. Switching on the furnace: Regulation of heat production in brown adipose tissue. Mol Asp Med. 2019;68(August):60–73.
Jeschke MG. The hepatic response to thermal injury: Is the liver important for postburn outcomes? Mol Med. 2009;15(9–10):337–51.
Cuyàs E, Verdura S, Micol V, Joven J, Bosch-Barrera J, Encinar JA, et al. Revisiting silibinin as a novobiocin-like Hsp90 C-terminal inhibitor: computational modeling and experimental validation. Food Chem Toxicol. 2019;132:110645.
Cypess AM, Weiner LS, Roberts-Toler C, Elía EF, Kessler SH, Kahn PA, et al. Activation of human brown adipose tissue by a β3-adrenergic receptor agonist. Cell Metab. 2015;21(1):33–8.
Lim S, Park J, Um JY. Ginsenoside Rb1 induces beta 3 adrenergic receptor–dependent lipolysis and thermogenesis in 3T3-L1 adipocytes and db/db mice. Front Pharmacol. 2019;10:1154.
Grujic D, Susulic VS, Harper M-E, Himms-Hagen J, Cunningham BA, Corkey BE, et al. 3-adrenergic receptors on white and brown adipocytes mediate 3-selective agonist-induced effects on energy expenditure, insulin secretion, and food intake: a study using transgenic and gene knockout mice*. J Biol Chem. 1997;272:17689.
Susulic VS, Frederich RC, Lawitts J, Tozzo E, Kahn BB, Harper M-E, et al. Targeted disruption of the 3-adrenergic receptor gene*. J Biol Chem. 1995;270:29483.
Arner P. Human fat cell lipolysis: biochemistry, regulation and clinical role. Best Pract Res Clin Endocrinol Metab. 2005;19:471–82.
Concha F, Prado G, Quezada J, Ramirez A, Bravo N, Flores C, et al. Nutritional and non-nutritional agents that stimulate white adipose tissue browning. Rev Endocr Metab Disord. 2019;20:161–71.
Seki T, Hosaka K, Lim S, Fischer C, Honek J, Yang Y, et al. Endothelial PDGF-CC regulates angiogenesis-dependent thermogenesis in beige fat. Nat Commun. 2016;7:1.
Guilherme A, Yenilmez B, Bedard AH, Henriques F, Liu D, Lee A, et al. Control of adipocyte thermogenesis and lipogenesis through β3-adrenergic and thyroid hormone signal integration. Cell Rep. 2020;31(5):107598.
Alcalá M, Calderon-Dominguez M, Serra D, Herrero L, Viana M. Mechanisms of impaired brown adipose tissue recruitment in obesity. Front Physiol. 2019;10:94.
Jimenez M, Barbatelli G, Allevi R, Cinti S, Seydoux J, Giacobino JP, et al. β3-adrenoceptor knockout in C57BL/6J mice depresses the occurrence of brown adipocytes in white fat. Eur J Biochem. 2003;270(4):699–705.
Herz CT, Kiefer FW. Adipose tissue browning in mice and humans. J Endocrinol. 2019;241(3):R97-109.
Evans SS, Repasky EA, Fisher DT. Fever and the thermal regulation of immunity: the immune system feels the heat. Nat Rev Immunol. 2015;15(6):335–49.
Castellani JW, Young AJ. Human physiological responses to cold exposure: acute responses and acclimatization to prolonged exposure. Auton Neurosci. 2016;196:63–74.
Gensini GF, Conti AA. The evolution of the concept of “fever” in the history of medicine: from pathological picture per se to clinical epiphenomenon (and vice versa). J Infect. 2004;49(2):85–7.
Garami A, Steiner AA, Romanovsky AA. Fever and hypothermia in systemic inflammation. Handb Clin Neurol. 2018;157(February):565–97.
Tateda K, Matsumoto T, Miyazaki S, Yamaguchi K. Lipopolysaccharide-induced lethality and cytokine production in aged mice. Infect Immun. 1996;64(3):769–74.
Copeland S, Shaw Warren H, Lowry SF, Galvano SE, Remick D. Acute inflammatory response to endotoxin in mice and humans. Clin Diagn Lab Immunol. 2005;12(1):60–7.
Zhao J, Bi W, Xiao S, Lan X, Cheng X, Zhang J, et al. Neuroinflammation induced by lipopolysaccharide causes cognitive impairment in mice. Sci Rep. 2019;9(1):5790.
Rudaya AY, Steiner AA, Robbins JR, Dragic AS, Romanovsky AA. Thermoregulatory responses to lipopolysaccharide in the mouse: dependence on the dose and ambient temperature. Am J Physiol Regul Integr Comp Physiol. 2005;289:1244–52.
Corrigan JJ, Fonseca MT, Flatow EA, Lewis K, Steiner AA. Hypometabolism and hypothermia in the rat model of endotoxic shock: Independence of circulatory hypoxia. J Physiol. 2014;592(17):3901–16.
Wanner SP, Yoshida K, Kulchitsky VA, Ivanov AI, Kanosue K, Romanovsky AA. Lipopolysaccharide-induced neuronal activation in the paraventricular and dorsomedial hypothalamus depends on ambient temperature. PLoS ONE. 2013;8(9):4–9.
Osaka T. Lipopolysaccharide-induced thermogenesis mediated by GABA in the preoptic area of anesthetized rats. J Therm Biol. 2006;31:229–34.
Tham S, Thompson R, Landeg O, Murray KA, Waite T. Indoor temperature and health: a global systematic review. Public Health. 2020;179:9–17.
Cheshire WP. Thermoregulatory disorders and illness related to heat and cold stress. Auton Neurosci. 2016;196:91–104.
Rumbus Z, Matics R, Hegyi P, Zsiboras C, Szabo I, Illes A, et al. Fever is associated with reduced, hypothermia with increased mortality in septic patients: a meta-analysis of clinical trials. PLoS ONE. 2017;12(1):1–15.
Fu SH, Gasparrini A, Rodriguez PS, Jha P. Mortality attributable to hot and cold ambient temperatures in India: a nationally representative case-crossover study. PLoS Med. 2018;15(7):1–17.
Chen R, Yin P, Wang L, Liu C, Niu Y, Wang W, et al. Association between ambient temperature and mortality risk and burden: time series study in 272 main Chinese cities. BMJ. 2018;363:k4306.
Lee SL, Battistella FD, Go K. Hypothermia induces T-cell production of immunosuppressive cytokines. J Surg Res. 2001;100(2):150–3.
Kurz A, Sessler DI, Lenhardt R. Perioperative normothermia to reduce the incidence of surgical-wound infection and shorten hospitalization. N Engl J Med. 1996;334(19):1209–15.
Hylander BL, Repasky EA. Thermoneutrality, mice, and cancer: a heated opinion. Trends Cancer. 2016;2(4):166–75.
Mace TA, Zhong L, Kilpatrick C, Zynda E, Lee C-T, Capitano M, et al. Differentiation of CD8 + T cells into effector cells is enhanced by physiological range hyperthermia. J Leukoc Biol. 2011;90(5):951–62.
Kokolus KM, Capitano ML, Lee CT, Eng JWL, Waight JD, Hylander BL, et al. Baseline tumor growth and immune control in laboratory mice are significantly influenced by subthermoneutral housing temperature. Proc Natl Acad Sci USA. 2013;110(50):20176–81.
Du G, Liu Y, Li J, Liu W, Wang Y, Li H. Hypothermic microenvironment plays a key role in tumor immune subversion. Int Immunopharmacol. 2013;17(2):245–53.
Dieing A, Ahlers O, Hildebrandt B, Kerner T, Tamm I, Possinger K, et al. The effect of induced hyperthermia on the immune system. Prog Brain Res. 2007;162:137–52.
Kobayashi Y, Ito Y, Ostapenko VV, Sakai M, Matsushita N, Imai K, et al. Fever-range whole-body heat treatment stimulates antigen-specific T-cell responses in humans. Immunol Lett. 2014;162(1):256–61.
Ostberg JR, Dayanc BE, Yuan M, Oflazoglu E, Repasky EA. Enhancement of natural killer (NK) cell cytotoxicity by fever-range thermal stress is dependent on NKG2D function and is associated with plasma membrane NKG2D clustering and increased expression of MICA on target cells. J Leukoc Biol. 2007;82(5):1322–31.
Umar D, Das A, Gupta S, Chattopadhyay S, Sarkar D, Mirji G, et al. Febrile temperature change modulates CD4 T cell differentiation via a TRPV channel-regulated Notch-dependent pathway. Proc Natl Acad Sci USA. 2020;117(36):22357–66.
Matsumoto K, Yamamoto N, Hagiwara S, Saito M, Furue H, Shigetomi T, et al. Optimization of hyperthermia and dendritic cell immunotherapy for squamous cell carcinoma. Oncol Rep. 2011;25(6):1525–32.
PubMed Google Scholar
Jackson TC, Kochanek PM. A new vision for therapeutic hypothermia in the era of targeted temperature management: a speculative synthesis. Ther Hypotherm Temp Manag. 2019;9(1):13–47.
Gu LJ, Xiong XX, Ito T, Lee J, Xu BH, Krams S, et al. Moderate hypothermia inhibits brain inflammation and attenuates stroke-induced immunodepression in rats. CNS Neurosci Ther. 2014;20(1):67–75.
Stanek A, Cholewka A, Wielkoszyński T, Romuk E, Sieroń A. Whole-body cryotherapy decreases the levels of inflammatory, oxidative stress, and atherosclerosis plaque markers in male patients with active-phase ankylosing spondylitis in the absence of classical cardiovascular risk factors. Mediators Inflamm. 2018;2018:1.
Lee JH, Wei ZZ, Cao W, Won S, Gu X, Winter M, et al. Regulation of therapeutic hypothermia on inflammatory cytokines, microglia polarization, migration and functional recovery after ischemic stroke in mice. Neurobiol Dis. 2016;96:248–60.
Wilson LJ, Dimitriou L, Hills FA, Gondek MB, Cockburn E. Whole body cryotherapy, cold water immersion, or a placebo following resistance exercise: a case of mind over matter? Eur J Appl Physiol. 2019;119(1):135–47.
Ziemann E, Olek RA, Grzywacz T, Kaczor JJ, Antosiewicz J, Skrobot W, et al. Whole-body cryostimulation as an effective way of reducing exercise-induced inflammation and blood cholesterol in young men. Eur Cytokine Netw. 2014;25(1):14–23.
Stanek A, Romuk E, Wielkoszyński T, Bartuś S, Cieślar G, Cholewka A. Decreased lipid profile and oxidative stress in healthy subjects who underwent whole-body cryotherapy in closed cryochamber with subsequent kinesiotherapy. Oxid Med Cell Longev. 2019;2019:1.
Coolbaugh CL, Damon BM, Bush EC, Welch EB, Towse TF. Cold exposure induces dynamic, heterogeneous alterations in human brown adipose tissue lipid content. Sci Rep. 2019;9(1):13600.
Sun YJ, Zhang ZY, Fan B, Li GY. Neuroprotection by therapeutic hypothermia. Front Neurosci. 2019;13:1–11.
Svedung Wettervik TM, Engquist H, Lenell S, Howells T, Hillered L, Rostami E, et al. Systemic hyperthermia in traumatic brain injury—relation to intracranial pressure dynamics, cerebral energy metabolism, and clinical outcome. J Neurosurg Anesthesiol. 2021;33(4):329–36.
Bain AR, Hoiland RL, Donnelly J, Nowak-Flück D, Sekhon M, Tymko MM, et al. Cerebral metabolism, oxidation and inflammation in severe passive hyperthermia with and without respiratory alkalosis. J Physiol. 2020;598(5):943–54.
Thorne AM, Ubbink R, Bruggenwirth IMA, Nijsten MW, Porte RJ, de Meijer VE. Hyperthermia-induced changes in liver physiology and metabolism: a rationale for hyperthermic machine perfusion. Am J Physiol Gastrointest Liver Physiol. 2020;319(1):G43-50.
Clapham JC. Central control of thermogenesis. Neuropharmacology. 2012;63(1):111–23.
Morrison SF. Central neural control of thermoregulation and brown adipose tissue. Auton Neurosci. 2016;196:14–24.
Fan G, Dang X, Li Y, Chen J, Zhao R, Yang X. Zinc-α2-glycoprotein promotes browning of white adipose tissue in cold-exposed male mice. Mol Cell Endocrinol. 2020;501:110669.
Luo X, Jia R, Zhang Q, Sun B, Yan J. Cold-Induced browning dynamically alters the expression profiles of inflammatory adipokines with tissue specificity in mice. Int J Mol Sci. 2016;17(5):795.
Article PubMed Central CAS Google Scholar
Reitman ML. Of mice and men—environmental temperature, body temperature, and treatment of obesity. FEBS Lett. 2018;592(12):2098–107.
Brychta RJ, Huang S, Wang J, Leitner BP, Hattenbach JD, Bell SL, et al. Quantification of the capacity for cold-induced thermogenesis in young men with and without obesity. J Clin Endocrinol Metab. 2019;104(10):4865–78.
Blauw LL, Aziz NA, Tannemaat MR, Blauw CA, de Craen AJ, Pijl H, et al. Diabetes incidence and glucose intolerance prevalence increase with higher outdoor temperature. BMJ Open Diabetes Res Care. 2017;5(1):e000317.
Kanazawa S. Does global warming contribute to the obesity epidemic? Environ Res. 2020;182:108962.
Li Y, Wang D, Ping X, Zhang Y, Zhang T, Wang L, et al. Local hyperthermia therapy induces browning of white fat and treats obesity. Cell. 2022;185(6):949-966.e19.
Patsouris D, Qi P, Abdullahi A, Stanojcic M, Chen P, Parousis A, et al. Burn induces browning of the subcutaneous white adipose tissue in mice and humans. Cell Rep. 2015;13(8):1538–44.
Vinaik R, Barayan D, Abdullahi A, Jeschke MG. NLRP3 inflammasome mediates white adipose tissue browning after burn. Am J Physiol Endocrinol Metab. 2019;317(5):E751–9.
Böstrom P, Wu JJM, et al. A PGC1- a -dependent myokine that drives brown-fat-like development of white fat and thermogenesis. Nature. 2012;481:463–8.
Roberts LD, Boström P, O’Sullivan JF, Schinzel RT, Lewis GD, Dejam A, et al. β-Aminoisobutyric acid induces browning of white fat and hepatic β-oxidation and is inversely correlated with cardiometabolic risk factors. Cell Metab. 2014;19(1):96–108.
Cao W, Daniel KW, Robidoux J, Puigserver P, Medvedev AV, Bai X, et al. p38 Mitogen-activated protein kinase is the central regulator of cyclic AMP-dependent transcription of the brown fat uncoupling protein 1 gene. Mol Cell Biol. 2004;24(7):3057–67.
Powers SK, Deminice R, Ozdemir M, Yoshihara T, Bomkamp MP, Hyatt H. Exercise-induced oxidative stress: friend or foe? J Sport Heal Sci. 2020;9(5):415–25.
Reddy A, Bozi LHM, Yaghi OK, Mills EL, Xiao H, Nicholson HE, et al. pH-gated succinate secretion regulates muscle remodeling in response to exercise. Cell. 2020;183(1):62-75.e17.
Nishimura T, Nakatake Y, Konishi M, Itoh N. Identification of a novel FGF, FGF-21, preferentially expressed in the liver. Biochim Biophys Acta Gene Struct Expr. 2000;1492(1):203–6.
Giralt M, Gavaldà-Navarro A, Villarroya F. Fibroblast growth factor-21, energy balance and obesity. Mol Cell Endocrinol. 2015;418:66–73.
Tacer KF, Bookout AL, Ding X, Kurosu H, John GB, Wang L, et al. Research resource: Comprehensive expression atlas of the fibroblast growth factor system in adult mouse. Mol Endocrinol. 2010;24(10):2050–64.
Kharitonenkov A, Jaskunas SR, Shanafelt AB, Shiyanova TL, Koester A, Ford AM, et al. FGF-21 as a novel metabolic regulator. J Clin Invest. 2005;115:1627.
Luo Y, Ye S, Li X, Lu W. Emerging structure-function paradigm of endocrine FGFs in metabolic diseases. Trends Pharmacol Sci. 2019;40:142–53.
Goetz R, Beenken A, Ibrahimi OA, Kalinina J, Olsen SK, Eliseenkova AV, et al. Molecular insights into the klotho-dependent, endocrine mode of action of fibroblast growth factor 19 subfamily members. Mol Cell Biol. 2007;27(9):3417–28.
Yang C, Jin C, Li X, Wang F, McKeehan WL, Luo Y. Differential specificity of endocrine FGF19 and FGF21 to FGFR1 and FGFR4 in complex with KLB. PLoS One. 2012;7(3):e33870.
Fisher FM, Maratos-Flier E. Understanding the physiology of FGF21. Annu Rev Physiol. 2016;78(1):223–41.
Martínez-Garza Ú, Torres-Oteros D, Yarritu-Gallego A, Marrero PF, Haro D, Relat J. Fibroblast growth factor 21 and the adaptive response to nutritional challenges. Int J Mol Sci. 2019;20:4692.
Dushay J, Chui PC, Gopalakrishnan GS, Varela-Rey M, Crawley M, Fisher FM, et al. Increased fibroblast growth factor 21 in obesity and nonalcoholic fatty liver disease. Gastroenterology. 2010;139(2):456–63.
Cuevas-Ramos DASCA. Modulation of energy balance by fibroblast growth factor 21. Hormone Mol Biol Clin Investig. 2016. https://doi.org/10.1515/hmbci-2016-0023 .
Oishi K, Uchida D, Ishida N. Circadian expression of FGF21 is induced by PPARα activation in the mouse liver. FEBS Lett. 2008;582(25–26):3639–42.
Lundåsen T, Hunt MC, Nilsson LM, Sanyal S, Angelin B, Alexson SEH, et al. PPARα is a key regulator of hepatic FGF21. Biochem Biophys Res Commun. 2007;360(2):437–40.
Gälman C, Lundåsen T, Kharitonenkov A, Bina HA, Eriksson M, Hafström I, et al. The circulating metabolic regulator FGF21 is induced by prolonged fasting and PPARα activation in man. Cell Metab. 2008;8(2):169–74.
Xu J, Lloyd DJ, Hale C, Stanislaus S, Chen M, Sivits G, et al. Fibroblast growth factor 21 reverses hepatic steatosis, increases energy expenditure, and improves insulin sensitivity in diet-induced obese mice. Diabetes. 2009;58(1):250–9.
Fazeli PK, Lun M, Kim SM, Bredella MA, Wright S, Zhang Y, et al. FGF21 and the late adaptive response to starvation in humans. J Clin Invest. 2015;125(12):4601–11.
Badman MK, Pissios P, Kennedy AR, Koukos G, Flier JS, Maratos-Flier E. Hepatic fibroblast growth factor 21 is regulated by PPARα and is a key mediator of hepatic lipid metabolism in ketotic states. Cell Metab. 2007;5(6):426–37.
Laeger T, Henagan TM, Albarado DC, Redman LM, Bray GA, Noland RC, et al. FGF21 is an endocrine signal of protein restriction. J Clin Invest. 2014;124(9):3913–22.
De Sousa-Coelho AL, Marrero PF, Haro D. Activating transcription factor 4-dependent induction of FGF21 during amino acid deprivation. Biochem J. 2012;443(1):165–71.
De Sousa-Coelho AL, Relat J, Hondares E, Pérez-Martí A, Ribas F, Villarroya F, et al. FGF21 mediates the lipid metabolism response to amino acid starvation. J Lipid Res. 2013;54(7):1786–97.
Muise ES, Azzolina B, Kuo DW, El-Sherbeini M, Tan Y, Yuan X, et al. Adipose fibroblast growth factor 21 is up-regulated by peroxisome proliferator-activated receptor γ and altered metabolic states. Mol Pharmacol. 2008;74(2):403–12.
Samms RJ, Murphy M, Fowler MJ, Cooper S, Emmerson P, Coskun T, et al. Dual effects of fibroblast growth factor 21 on hepatic energy metabolism. J Endocrinol. 2015;227(1):37–47.
Lin Z, Tian H, Lam KSL, Lin S, Hoo RCL, Konishi M, et al. Adiponectin mediates the metabolic effects of FGF21 on glucose homeostasis and insulin sensitivity in mice. Cell Metab. 2013;17(5):779–89.
Holland WL, Adams AC, Brozinick JT, Bui HH, Miyauchi Y, Kusminski CM, et al. An FGF21-adiponectin-ceramide axis controls energy expenditure and insulin action in mice. Cell Metab. 2013;17(5):790–7.
Fisher FF, Kleiner S, Douris N, Fox EC, Mepani RJ, Verdeguer F, et al. FGF21 regulates PGC-1α and browning of white adipose tissues in adaptive thermogenesis. Genes Dev. 2012;26(3):271–81.
Pérez-Martí A, Sandoval V, Marrero PF, Haro D, Relat J. Nutritional regulation of fibroblast growth factor 21: from macronutrients to bioactive dietary compounds. Hormone Mol Biol Clin Investig. 2017. https://doi.org/10.1515/hmbci-2016-0034 .
Brent GA. Mechanisms of thyroid hormone action. J Clin Invest. 2012;122:3035–43.
Gereben B, Zavacki AM, Ribich S, Kim BW, Huang SA, Simonides WS, et al. Cellular and molecular basis of deiodinase-regulated thyroid hormone signaling. Endocr Rev. 2008;29:898–938.
Mullur R, Liu YY, Brent GA. Thyroid hormone regulation of metabolism. Physiol Rev. 2014;94(2):355–82.
Rubio IG, Medeiros-Neto G. Mutations of the thyroglobulin gene and its relevance to thyroid disorders. Curr Opin Endocrinol Diabetes Obes. 2009;16(5):373–8.
Zimmermann MB. Iodine deficiency. Endocr Rev. 2009;30:376–408.
Carvalho DP, Dupuy C. Thyroid hormone biosynthesis and release. Mol Cell Endocrinol. 2017;458:6–15.
Cheng SY, Leonard JL, Davis PJ. Molecular aspects of thyroid hormone actions. Endocr Rev. 2010;31:139–70.
Ortiga-Carvalho TM, Sidhaye AR, Wondisford FE. Thyroid hormone receptors and resistance to thyroid hormone disorders. Nat Rev Endocrinol. 2014;10:582–91.
Lindsey RC, Mohan S. Thyroid hormone acting via TRβ induces expression of browning genes in mouse bone marrow adipose tissue. Endocrine. 2017;56(1):109–20.
Haber RS, Loeb JN. Stimulation of potassium efflux in rat liver by a low dose of thyroid hormone: evidence for enhanced cation permeability in the absence of Na. K-ATPase Induct Endocrinol. 1986;118(1):207–11.
Silva JE. Thermogenic mechanisms and their hormonal regulation. Physiol Rev. 2006;86:435–64.
Freake HC, Schwartz HL, Oppenheimer JH. The regulation of lipogenesis by thyroid hormone and its contribution to thermogenesis. Endocrinology. 1989;125(6):2868–74.
Clausen T, Van Hardeveld C, Everts ME. Significance of cation transport in control of energy metabolism and thermogenesis. Physiol Rev. 1991;71:733–74.
Barbe P, Larrouy D, Boulanger C, Chevillotte E, Viguerie N, Thalamas C, et al. Triiodothyronine-mediated upregulation of UCP2 and UCP3 mRNA expression in human skeletal muscle without coordinated induction of mitochondrial respiratory chain genes. FASEB J. 2001;15(1):13–5.
Liu YY, Schultz JJ, Brent GA. A thyroid hormone receptor α gene mutation (P398H) is associated with visceral adiposity and impaired catecholamine-stimulated lipolysis in mice. J Biol Chem. 2003;278(40):38913–20.
Lee JY, Takahashi N, Yasubuchi M, Kim Y, Hashizaki H, Kim MJ, et al. Triiodothyronine induces ucp-1 expression and mitochondrial biogenesis in human adipocytes. Am J Physiol Cell Physiol. 2012;302(2):c463.
Weiner J, Hankir M, Heiker JT, Fenske W, Krause K. Thyroid hormones and browning of adipose tissue. Mol Cell Endocrinol. 2017;458:156–9.
López M, Varela L, Vázquez MJ, Rodríguez-Cuenca S, González CR, Velagapudi VR, et al. Hypothalamic AMPK and fatty acid metabolism mediate thyroid regulation of energy balance. Nat Med. 2010;16(9):1001–8.
Bianco AC, Sheng X, Silva JE. Triiodothyronine amplifies norepinephrine stimulation of uncoupling protein gene transcription by a mechanism not requiring protein synthesis*. J Biol Chem. 1988;263:18168.
Martínez-Sánchez N, Moreno-Navarrete JM, Contreras C, Rial-Pensado E, Fernø J, Nogueiras R, et al. Thyroid hormones induce browning of white fat. J Endocrinol. 2017;232(2):351–62.
Cipolla-Neto J, Amaral FG, Afeche SC, Tan DX, Reiter RJ. Melatonin, energy metabolism, and obesity: a review. J Pineal Res. 2014;56(4):371–81.
Zhao D, Yu Y, Shen Y, Liu Q, Zhao Z, Sharma R, et al. Melatonin synthesis and function: evolutionary history in animals and plants. Front Endocrinol (Lausanne). 2019;17(10):249.
Elmquist JK, Bjorbaek C, Ahima RS, Flier JS, Saper CB. Distributions of leptin receptor mRNA isoforms in the rat brain. J Comp Neurol. 1998;395(4):535–47.
Hsuchou H, Kastin AJ, Tu H, Markadakis EN, Stone KP, Wang Y, et al. Effects of cell-type specific leptin receptor mutation on leptin transport across the BBB. Peptides. 2011;32(7):1392–9.
Hsuchou H, Wang Y, Cornelissen-Guillaume GG, Kastin AJ, Jang E, Halberg F, et al. Diminished leptin signaling can alter circadian rhythm of metabolic activity and feeding. J Appl Physiol. 2013;115(7):995–1003.
Siegrist-Kaiser CA, Pauli V, Juge-Aubry CE, Boss O, Pernin A, Chin WW, et al. Direct effects of leptin on brown and white adipose tissue. J Clin Invest. 1997;100(11):2858.
Hoffmann A, Ebert T, Hankir MK, Flehmig G, Klöting N, Jessnitzer B, et al. Leptin improves parameters of brown adipose tissue thermogenesis in lipodystrophic mice. Nutrients. 2021;13(8):1–10.
Van Den Berg R, Kooijman S, Noordam R, Van Heemst D, Biermasz NR, Rensen PCN. A diurnal rhythm in brown adipose tissue causes rapid clearance and combustion of plasma lipids at wakening. Cell Rep. 2018;22:3521–33.
Matsushita M, Nirengi S, Hibi M, Wakabayashi H, Lee S, Domichi M, et al. Diurnal variations of brown fat thermogenesis and fat oxidation in humans. Int J Obes. 2021;45(11):2499–505.
Halpern B, Mancini MC, Bueno C, Barcelos IP, De Melo ME, Lima MS, et al. Melatonin increases brown adipose tissue volume and activity in patients with melatonin deficiency: a proof-of-concept study. Diabetes. 2019;68(5):947–52.
Halpern B, Mancini MC, Mendes C, MacHado CML, Prando S, Sapienza MT, et al. Melatonin deficiency decreases brown adipose tissue acute thermogenic capacity of in rats measured by 18F-FDG PET. Diabetol Metab Syndr. 2020;12(1):1–7.
Agil A, Navarro-Alarcon M, Ali FAZ, Albrakati A, Salagre D, Campoy C, et al. Melatonin enhances the mitochondrial functionality of brown adipose tissue in obese-diabetic rats. Antioxidants (Basel, Switzerland). 2021;10(9):1482.
Wang S, Liang X, Yang Q, et al. Resveratrol induces brown-like adipocyte formation in white fat through activation of AMP-activated protein kinase (AMPK) α1. Int J Obes. 2015;39(6):967–76.
Choi JH, Kim SW, Yu R, Yun JW. Monoterpene phenolic compound thymol promotes browning of 3T3 - L1 adipocytes. Eur J Nutr. 2016;56(7):2329–41.
Kang N, Mukherjee S, Yun J. Trans-cinnamic acid stimulates white fat browning. Nutrients. 2019;11(3):577.
Article CAS PubMed Central Google Scholar
Lee SG, Parks JS, Kang HW. Quercetin, a functional compound of onion peel, remodels white adipocytes to brown-like adipocytes. J Nutr Biochem. 2017;2016(46):62–71.
Arias N, Picó C, Teresa Macarulla M, Oliver P, Miranda J, Palou A, et al. A combination of resveratrol and quercetin induces browning in white adipose tissue of rats fed an obesogenic diet. Obes (Silver Spring). 2016;25:111–21.
Choi JH, Yun JW. Chrysin induces brown fat-like phenotype and enhances lipid metabolism in 3T3-L1 adipocytes. Nutrition. 2016;32:1002–10.
Baskaran P, Krishnan V, Ren J, Thyagarajan B. Capsaicin induces browning of white adipose tissue and counters obesity by activating TRPV1 channel-dependent mechanisms. Br J Pharmacol. 2016;173(15):2369–89.
Jiang C, Zhai M, Yan D, Li D, Li C, Zhang Y. Dietary menthol-induced TRPM8 activation enhances WAT “ browning ” and ameliorates diet-induced obesity. Oncotarget. 2017;8(43):75114–26.
Maeda H, Hosokawa M, Sashima T, Funayama K, Miyashita K. Fucoxanthin from edible seaweed, Undaria pinnatifida, shows antiobesity effect through UCP1 expression in white adipose tissues. Biochem Biophys Res Commun. 2005;332:392–7.
Serra F, Bonet ML, Puigserver P, Oliver J, Palou A. Stimulation of uncoupling protein 1 expression in brown adipocytes by naturally occurring carotenoids. Int J Obes Relat Metab Disord. 1999;23:650–6.
Chou Y, Ho C, Pan M. Immature Citrus reticulata extract promotes browning of beige adipocytes in high-fat diet-induced C57BL / 6 mice. Food Chem. 2018;66:9769–9703.
Zhang Z, Zhang H, Li B, Meng X, Wang J, Zhang Y, et al. Berberine activates thermogenesis in white and brown adipose tissue. Nat Commun. 2014;5(1):1.
Neyrinck AM, Bindels LB, Geurts L, Van M, Cani PD, Delzenne NM. A polyphenolic extract from green tea leaves activates fat browning in high-fat-diet-induced obese mice. J Nutr Biochem. 2017;49:15–21.
Chen L, Chien Y, Liang C, Chan C, Huang H, Chien Y, et al. Green tea extract induces genes related to browning of white adipose tissue and limits weight-gain in high energy diet-fed rat. Food Nutr Res. 2017;61(1):1347480.
Palacios-gonzález B, Vargas-castillo A, Velázquez-villegas LA, Vasquez-reyes S, López P, Noriega LG, et al. ScienceDirect genistein increases the thermogenic program of subcutaneous WAT and increases energy expenditure in mice*. J Nutr Biochem. 2019;68:59–68.
Parray HA, Lone J, Park JP, Choi JW, Yun J. Magnolol promotes thermogenesis and attenuates oxidative stress in 3T3-L1 adipocytes. Nutrition. 2018;50:82–90.
Lone J, Yun JW. Honokiol exerts dual effects on browning and apoptosis of adipocytes. Pharmacol Reports. 2017;69(6):1357–65.
Mu Q, Fang X, Li X, Zhao D, Mo F, Jiang G, et al. Ginsenoside Rb1 promotes browning through regulation of PPAR g in 3T3-L1 adipocytes. Biochem Biophys Res Commun. 2015;466(3):530–5.
Lee K, Seo Y, Song J, Lee B. Ginsenoside Rg1 promotes browning by inducing UCP1 expression and mitochondrial activity in 3T3-L1 and subcutaneous white adipocytes. J Ginseng Res. 2018;43(4):589–99.
Bargut TC, Silva-e-Silva AC, Souza-Mello V, Mandarim-de-Lacerda CAAM, Mandarim A. Mice fed fish oil diet and upregulation of brown adipose tissue thermogenic markers. Eur J Nutr. 2016;55(1):159–69.
Pahlavani M, Razafimanjato F, Ramalingam L, Nishan S. Eicosapentaenoic acid regulates brown adipose tissue metabolism in high-fat-fed mice and in clonal brown adipocytes. J Nutr Biochem. 2016;39:101–9.
Kim M, Goto T, Yu R, Uchida K, Tominaga M, Kano Y. Fish oil intake induces UCP1 upregulation in brown and white adipose tissue via the sympathetic nervous system. Sci Rep. 2015;5:1–12.
Shen W, Chuang C, Martinez K, Reid T, Brown JM, Xi L, et al. Conjugated linoleic acid reduces adiposity and increases markers of browning and infl ammation in white adipose tissue of mice. J Lipid Res. 2013;54(4):909–22.
Mozaffarian D. Dietary and policy priorities for cardiovascular disease, diabetes, and obesity. Circulation. 2016;133(2):187–225.
Hofer SJ, Carmona-Gutierrez D, Mueller MI, Madeo F. The ups and downs of caloric restriction and fasting: from molecular effects to clinical application. EMBO Mol Med. 2022;14(1):e14418.
Weindruch R, Sohal RS. Caloric intake and aging. N Engl J Med. 1997;337(14):986–94.
Fabbiano S, Suárez-Zamorano N, Rigo D, Veyrat-Durebex C, Stevanovic Dokic A, Colin DJ, et al. Caloric restriction leads to browning of white adipose tissue through type 2 immune signaling. Cell Metab. 2016;24(3):434–46.
Anton SD, Moehl K, Donahoo WT, Marosi K, Lee SA, Mainous AG, et al. Flipping the metabolic switch: understanding and applying the health benefits of fasting. Obesity. 2018;26(2):254–68.
Patel S, Alvarez-Guaita A, Melvin A, Rimmington D, Dattilo A, Miedzybrodzka EL, et al. GDF15 provides an endocrine signal of nutritional stress in mice and humans. Cell Metab. 2019;29(3):707-718.e8.
de Cabo R, Mattson MP. Effects of intermittent fasting on health, aging, and disease. N Engl J Med. 2019;381(26):2541–51.
Liu B, Hutchison AT, Thompson CH, Lange K, Heilbronn LK. Markers of adipose tissue inflammation are transiently elevated during intermittent fasting in women who are overweight or obese. Obes Res Clin Pract. 2019;13(4):408–15.
Liu B, Page AJ, Hutchison AT, Wittert GA, Heilbronn LK. Intermittent fasting increases energy expenditure and promotes adipose tissue browning in mice. Nutrition. 2019;1(66):38–43.
de Marinho TS, Ornellas F, Aguila MB, Mandarim-de-Lacerda CA. Browning of the subcutaneous adipocytes in diet-induced obese mouse submitted to intermittent fasting. Mol Cell Endocrinol. 2020;513:110872.
Shinoda K, Luijten IHN, Hasegawa Y, Hong H, Sonne SB, Kim M, et al. Genetic and functional characterization of clonally derived adult human brown adipocytes. Nat Med. 2015;21(4):389–94.
Xue R, Lynes MD, Dreyfuss JM, Shamsi F, Schulz TJ, Zhang H, et al. Clonal analyses and gene profiling identify genetic biomarkers of the thermogenic potential of human brown and white preadipocytes. Nat Med. 2015;21(7):760–8.
Zuriaga MA, Fuster JJ, Gokce N, Walsh K. Humans and mice display opposing patterns of “browning” gene expression in visceral and subcutaneous white adipose tissue depots. Front Cardiovasc Med. 2017;5:4.
Li G, Xie C, Lu S, Nichols RG, Tian Y, Li L, et al. Intermittent fasting promotes white adipose browning and decreases obesity by shaping the gut microbiota. Cell Metab. 2017;26(4):672-685.e4.
von Schwartzenberg RJ, Bisanz JE, Lyalina S, Spanogiannopoulos P, Ang QY, Cai J, et al. Caloric restriction disrupts the microbiota and colonization resistance. Nat. 2021;595(7866):272–7.
Garretson JT, Szymanski LA, Schwartz GJ, Xue B, Ryu V, Bartness TJ. Lipolysis sensation by white fat afferent nerves triggers brown fat thermogenesis. Mol Metab. 2016;5(8):626–34.
Simcox J, Geoghegan G, Maschek JA, Bensard CL, Pasquali M, Miao R, et al. Global analysis of plasma lipids identifies liver-derived acylcarnitines as a fuel source for brown fat thermogenesis. Cell Metab. 2017;26(3):509-522.e6.
Schlein C, Talukdar S, Heine M, Fischer AW, Krott LM, Nilsson SK, et al. FGF21 lowers plasma triglycerides by accelerating lipoprotein catabolism in white and brown adipose tissues. Atherosclerosis. 2016;1(252):e239–40.
Berbeé JFP, Boon MR, Khedoe PPSJ, Bartelt A, Schlein C, Worthmann A, et al. Brown fat activation reduces hypercholesterolaemia and protects from atherosclerosis development. Nat Commun. 2015;6(1):1–11.
Lefebvre P, Cariou B, Lien F, Kuipers F, Staels B. Role of bile acids and bile acid receptors in metabolic regulation. Physiol Rev. 2009;89(1):147–91.
Watanabe M, Houten SM, Mataki C, Christoffolete MA, Kim BW, Sato H, et al. Bile acids induce energy expenditure by promoting intracellular thyroid hormone activation. Nat. 2006;439(7075):484–9.
Broeders EPM, Nascimento EBM, Havekes B, Brans B, Roumans KHM, Tailleux A, et al. The bile acid chenodeoxycholic acid increases human brown adipose tissue activity. Cell Metab. 2015;22(3):418–26.
Zietak M, Kozak LP. Bile acids induce uncoupling protein 1-dependent thermogenesis and stimulate energy expenditure at thermoneutrality in mice. Am J Physiol Endocrinol Metab. 2016;310(5):E346–54.
Fan M, Wang Y, Jin L, Fang Z, Peng J, Tu J, et al. Bile acid-mediated activation of brown fat protects from alcohol-induced steatosis and liver injury in mice. Cell Mol Gastroenterol Hepatol. 2022;13(3):809–26.
Zhou W, VanDuyne P, Zhang C, Riessen R, Barragan M, Rowitz BM, et al. Bile acid excess impairs thermogenic function in brown adipose tissue. bioRxiv. 2020;155:213.
Mu Y, Gudey SK, Landström M. Non-Smad signaling pathways. Cell Tissue Res. 2012;347(1):11–20.
Schmierer B, Hill CS. TGFβ–SMAD signal transduction: molecular specificity and functional flexibility. Nat Rev Mol Cell Biol. 2007;8(12):970–82.
Qian S, Tang Y, Tang Q-Q. Adipose tissue plasticity and the pleiotropic roles of BMP signaling. J Biol Chem. 2021;1(296):100678.
Tang QQ, Otto TC, Lane MD. Commitment of C3H10T1/2 pluripotent stem cells to the adipocyte lineage. Proc Natl Acad Sci USA. 2004;101(26):9607–11.
Qian S-W, Tang Y, Li X, Liu Y, Zhang Y-Y, Huang H-Y, et al. BMP4-mediated brown fat-like changes in white adipose tissue alter glucose and energy homeostasis. Proc Natl Acad Sci USA. 2013;110(9):E798-807.
Ezkurdia I, Juan D, Rodriguez JM, Frankish A, Diekhans M, Harrow J, et al. Multiple evidence strands suggest that there may be as few as 19,000 human protein-coding genes. Hum Mol Genet. 2014;23(22):5866–78.
Ong CT, Corces VG. Enhancer function: new insights into the regulation of tissue-specific gene expression. Nat Rev Genet. 2011;12(4):283–93.
Kajimura S, Seale P, Kubota K, Lunsford E, Frangioni JV, Gygi SP, et al. Initiation of myoblast to brown fat switch by a PRDM16–C/EBP-β transcriptional complex. Nature. 2009;460(7259):1154–8.
Ma X, Wang D, Zhao W, Xu L. Deciphering the roles of PPARγ in adipocytes via dynamic change of transcription complex. Front Endocrinol (Lausanne). 2018;9:1–10.
Lehrke M, Lazar MA. The many faces of PPARγ. Cell. 2005;123(6):993–9.
Madsen MS, Siersbæk R, Boergesen M, Nielsen R, Mandrup S. Peroxisome proliferator-activated receptor γ and C/EBPα synergistically activate key metabolic adipocyte genes by assisted loading. Mol Cell Biol. 2014;34(6):939–54.
Lefterova MI, Zhang Y, Steger DJ, Schupp M, Schug J, Cristancho A, et al. PPARγ and C/EBP factors orchestrate adipocyte biology via adjacent binding on a genome-wide scale. Genes Dev. 2008;22(21):2941–52.
Kroon T, Harms M, Maurer S, Bonnet L, Alexandersson I, Lindblom A, et al. PPARγ and PPARα synergize to induce robust browning of white fat in vivo. Mol Metab. 2020;36(February):1–14.
Rachid TL, Silva-Veiga FM, Graus-Nunes F, Bringhenti I, Mandarim-de-Lacerda CA, Souza-Mello V. Differential actions of PPAR-α and PPAR-β/δ on beige adipocyte formation: a study in the subcutaneous white adipose tissue of obese male mice. PLoS ONE. 2018;13(1):e0191365.
Ohno H, Shinoda K, Spiegelman BM, Kajimura S. PPAR agonists Induce a White-to-Brown fat conversion through stabilization of PRDM16 protein. Cell Metab. 2012;15(3):395–404.
Ishibashi J, Seale P. Functions of Prdm16 in thermogenic fat cells. Temperature. 2015;2(1):65–72.
Seale P, Conroe HM, Estall J, Kajimura S, Frontini A, Ishibashi J, et al. Prdm16 determines the thermogenic program of subcutaneous white adipose tissue in mice. J Clin Invest. 2011;121(1):96–105.
Cohen P, Levy JD, Zhang Y, Frontini A, Kolodin DP, Svensson KJ, et al. Ablation of PRDM16 and beige adipose causes metabolic dysfunction and a subcutaneous to visceral fat switch. Cell. 2014;156(1–2):304–16.
Harms MJ, Ishibashi J, Wang W, Lim HW, Goyama S, Sato T, et al. Prdm16 is required for the maintenance of brown adipocyte identity and function in adult mice. Cell Metab. 2014;19(4):593–604.
Seale P, Kajimura S, Yang W, Chin S, Rohas LM, Uldry M, et al. Transcriptional control of brown fat determination by PRDM16. Cell Metab. 2007;6(1):38–54.
Liang H, Ward WF. PGC-1α: a key regulator of energy metabolism. Am J Physiol Adv Physiol Educ. 2006;30(4):145–51.
Austin S, St-Pierre J. PGC1α and mitochondrial metabolism - emerging concepts and relevance in ageing and neurodegenerative disorders. J Cell Sci. 2012;125(21):4963–71.
Uguccioni G, Hood DA. The importance of PGC-1α in contractile activity-induced mitochondrial adaptations. Am J Physiol Endocrinol Metab. 2011;300(2):361–71.
Correia JC, Ferreira DMS, Ruas JL. Intercellular: Local and systemic actions of skeletal muscle PGC-1s. Trends Endocrinol Metab. 2015;26(6):305–14.
Lin J, Handschin C, Spiegelman BM. Metabolic control through the PGC-1 family of transcription coactivators. Cell Metab. 2005;1(6):361–70.
Wu Z, Puigserver P, Andersson U, Zhang C, Adelmant G, Mootha V, et al. Mechanisms controlling mitochondrial biogenesis and respiration through the thermogenic coactivator PGC-1. Cell. 1999;98(1):115–24.
Christianson JL, Boutet E, Puri V, Chawla A, Czech MP. Identification of the lipid droplet targeting domain of the Cidea protein. J Lipid Res. 2010;51(12):3455–62.
Puri V, Ranjit S, Konda S, Nicoloro SMC, Straubhaar J, Chawla A, et al. Cidea is associated with lipid droplets and insulin sensitivity in humans. Proc Natl Acad Sci USA. 2008;105(22):7833–8.
Reynolds TH, Banerjee S, Sharma VM, Donohue J, Couldwell S, Sosinsky A, et al. Effects of a high fat diet and voluntary wheel running exercise on cidea and cidec expression in liver and adipose tissue of mice. PLoS One. 2015;10(7):e0130259.
Jash S, Banerjee S, Lee MJ, Farmer SR, Puri V. CIDEA transcriptionally regulates UCP1 for britening and thermogenesis in human fat cells. iScience. 2019;20:73–89.
Dempersmier J, Sambeat A, Gulyaeva O, Paul SM, Hudak CSS, Raposo HF, et al. Cold-inducible Zfp516 activates UCP1 transcription to promote browning of white fat and development of brown fat. Mol Cell. 2015;57(2):235–46.
Ma X, Xu L, Alberobello AT, Gavrilova O, Bagattin A, Skarulis M, et al. Celastrol protects against obesity and metabolic dysfunction through activation of a HSF1-PGC1α transcriptional axis. Cell Metab. 2015;22(4):695–708.
Liu J, Lee J, Hernandez MAS, Mazitschek R, Ozcan U. Treatment of obesity with celastrol. Cell. 2015;161(5):999–1011.
Eguchi J, Wang X, Yu S, Kershaw EE, Chiu PC, Dushay J, et al. Transcriptional control of adipose lipid handling by IRF4. Cell Metab. 2011;13(3):249–59.
Kong X, Banks A, Liu T, Kazak L, Rao RR, Cohen P, et al. IRF4 is a key thermogenic transcriptional partner of PGC-1α. Cell. 2014;158(1):69–83.
Hiraike Y, Waki H, Yu J, Nakamura M, Miyake K, Nagano G, et al. NFIA co-localizes with PPARγ and transcriptionally controls the brown fat gene program. Nat Cell Biol. 2017;19(9):1081–92.
Rajakumari S, Wu J, Ishibashi J, Lim HW, Giang AH, Won KJ, et al. EBF2 determines and maintains brown adipocyte identity. Cell Metab. 2013;17(4):562–74.
Zhao XY, Li S, Wang GX, Yu Q, Lin JD. A long noncoding RNA transcriptional regulatory circuit drives thermogenic adipocyte differentiation. Mol Cell. 2014;55(3):372–82.
Stine RR, Shapira SN, Lim HW, Ishibashi J, Harms M, Won KJ, et al. EBF2 promotes the recruitment of beige adipocytes in white adipose tissue. Mol Metab. 2015;5(1):57–65.
Shao M, Ishibashi J, Kusminski CM, Wang QA, Hepler C, Vishvanath L, et al. Zfp423 maintains white adipocyte identity through suppression of the beige cell thermogenic gene program. Cell Metab. 2016;23(6):1167–84.
Shao M, Zhang Q, Truong A, Shan B, Vishvanath L, Li L, et al. ZFP423 controls EBF2 coactivator recruitment and PPARγ occupancy to determine the thermogenic plasticity of adipocytes. Genes Dev. 2021;35(21–22):1461–74.
Villanueva CJ, Waki H, Godio C, Nielsen R, Chou WL, Vargas L, et al. TLE3 is a dual-function transcriptional coregulator of adipogenesis. Cell Metab. 2011;13(4):413–27.
Pearson S, Loft A, Rajbhandari P, Simcox J, Lee S, Tontonoz P, et al. Loss of TLE3 promotes the mitochondrial program in beige adipocytes and improves glucose metabolism. Genes Dev. 2019;33(13–14):747–62.
Inagaki T, Sakai J, Kajimura S. Transcriptional and epigenetic control of brown and beige adipose cell fate and function. Nat Rev Mol Cell Biol. 2016;17(8):480–95.
Wang W, Seale P. Control of brown and beige fat development. Nat Rev Mol Cell Biol. 2016;17(11):691–702.
Loft A, Forss I, Mandrup S. Genome-wide insights into the development and function of thermogenic adipocytes. Trends Endocrinol Metab. 2017;28(2):104–20.
Squillaro T, Peluso G, Galderisi U, Di Bernardo G. Long non-coding RNAs in regulation of adipogenesis and adipose tissue function. Elife. 2020;1(9):1–15.
Nanduri R. Epigenetic regulators of white adipocyte browning. Epigenomes. 2021;5(1):3.
Ong BX, Brunmeir R, Zhang Q, Peng X, Idris M, Liu C, et al. Regulation of thermogenic adipocyte differentiation and adaptive thermogenesis through histone acetylation. Front Endocrinol (Lausanne). 2020;27(11):95.
Yamauchi T, Oike Y, Kamon J, Waki H, Komeda K, Tsuchida A, et al. Increased insulin sensitivity despite lipodystrophy in Crebbp heterozygous mice. Nat Genet. 2002;30(2):221–6.
Roh HC, Tsai LTY, Shao M, Tenen D, Shen Y, Kumari M, et al. Warming induces significant reprogramming of beige, but not brown. Adipocyte Cell Identity Cell Metab. 2018;27(5):1121-1137.e5.
CAS PubMed Google Scholar
Jin Q, Wang C, Kuang X, Feng X, Sartorelli V, Ying H, et al. Gcn5 and PCAF regulate PPARγ and Prdm16 expression to facilitate brown adipogenesis. Mol Cell Biol. 2014;34(19):3746–53.
Li F, Wu R, Cui X, Zha L, Yu L, Shi H, et al. Histone deacetylase 1 (HDAC1) negatively regulates thermogenic program in brown adipocytes via coordinated regulation of histone H3 lysine 27 (H3K27) deacetylation and methylation. J Biol Chem. 2016;291(9):4523–36.
Ferrari A, Longo R, Fiorino E, Silva R, Mitro N, Cermenati G, et al. HDAC3 is a molecular brake of the metabolic switch supporting white adipose tissue browning. Nat Commun. 2017;8(1):1.
Chatterjee TK, Basford JE, Knoll E, Tong WS, Blanco V, Blomkalns AL, et al. HDAC9 knockout mice are protected from adipose tissue dysfunction and systemic metabolic disease during high-fat feeding. Diabetes. 2014;63(1):176–87.
Bagchi RA, Ferguson BS, Stratton MS, Hu T, Cavasin MA, Sun L, et al. HDAC11 suppresses the thermogenic program of adipose tissue via BRD2. JCI Insight. 2018;3(15):120159–120159.
Mayoral R, Osborn O, McNelis J, Johnson AM, Oh DY, Izquierdo CL, et al. Adipocyte SIRT1 knockout promotes PPARγ activity, adipogenesis and insulin sensitivity in chronic-HFD and obesity. Mol Metab. 2015;4(5):378–91.
Thakore PI, Black JB, Hilton IB, Gersbach CA. Editing the epigenome: technologies for programmable transcription and epigenetic modulation. Nat Methods. 2016;13(2):127–37.
Lee J, Saha PK, Yang QH, Lee S, Jung YP, Suh Y, et al. Targeted inactivation of MLL3 histone H3-Lys-4 methyltransferase activity in the mouse reveals vital roles for MLL3 in adipogenesis. Proc Natl Acad Sci USA. 2008;105(49):19229–34.
Ohno H, Shinoda K, Ohyama K, Sharp LZ, Kajimura S. EHMT1 controls brown adipose cell fate and thermogenesis through the PRDM16 complex. Nat. 2013;504(7478):163–7.
Duteil D, Tosic M, Willmann D, Georgiadi A, Kanouni T, Schüle R. Lsd1 prevents age-programed loss of beige adipocytes. Proc Natl Acad Sci USA. 2017;114(20):5265–70.
Duteil D, Metzger E, Willmann D, Karagianni P, Friedrichs N, Greschik H, et al. LSD1 promotes oxidative metabolism of white adipose tissue. Nat Commun. 2014;5(1):1–14.
Zeng X, Jedrychowski MP, Chen Y, Serag S, Lavery GG, Gygi SP, et al. Lysine-specific demethylase 1 promotes brown adipose tissue thermogenesis via repressing glucocorticoid activation. Genes Dev. 2016;30(16):1822–36.
Abe Y, Rozqie R, Matsumura Y, Kawamura T, Nakaki R, Tsurutani Y, et al. JMJD1A is a signal-sensing scaffold that regulates acute chromatin dynamics via SWI/SNF association for thermogenesis. Nat Commun. 2015;6(1):1–14.
Deaton AM, Bird A. CpG islands and the regulation of transcription. Genes Dev. 2011;25(10):1010–22.
Shapira SN, Seale P. Transcriptional control of brown and beige fat development and function. Obesity (Silver Spring). 2019;27(1):13–21.
Kadam S, Emerson EBM. Each of these complexes contains one of two ATPases, BRG1 or BRM, and a variable subunit composition of BRG1-associated factors (BAFs). In Mol Cell. 1996;2003(11):377–89.
O’Brien J, Hayder H, Zayed Y, Peng C. Overview of microRNA biogenesis, mechanisms of actions, and circulation. Front Endocrinol (Lausanne). 2018;9:402.
Ng R, Hussain NA, Zhang Q, Chang C, Li H, Fu Y, et al. miRNA-32 drives brown fat thermogenesis and trans-activates subcutaneous white fat browning in mice. Cell Rep. 2017;19(6):1229–46.
Virtue AT, McCright SJ, Wright JM, Jimenez MT, Mowel WK, Kotzin JJ, et al. The gut microbiota regulates white adipose tissue inflammation and obesity via a family of microRNAs. Sci Transl Med. 2019;11(496):eaav1892–eaav1892.
Acharya A, Berry DC, Zhang H, Jiang Y, Jones BT, Hammer RE, et al. MiR-26 suppresses adipocyte progenitor differentiation and fat production by targeting Fbxl19. Genes Dev. 2019;33(19–20):1367–80.
Mori M, Nakagami H, Rodriguez-Araujo G, Nimura K, Kaneda Y. Essential role for miR-196a in brown adipogenesis of white fat progenitor cells. PLOS Biol. 2012;10(4):e1001314.
Hu F, Wang M, Xiao T, Yin B, He L, Meng W, et al. miR-30 promotes thermogenesis and the development of beige fat by targeting RIP140. Diabetes. 2015;64(6):2056–68.
Chou CF, Lin YY, Wang HK, Zhu X, Giovarelli M, Briata P, et al. KSRP ablation enhances brown fat gene program in white adipose tissue through reduced mir-150 expression. Diabetes. 2014;63(9):2949–61.
Fu T, Seok S, Choi S, Huang Z, Suino-Powell K, Xu HE, et al. MicroRNA 34a inhibits beige and brown fat formation in obesity in part by suppressing adipocyte fibroblast growth factor 21 signaling and SIRT1 function. Mol Cell Biol. 2014;34(22):4130–42.
Chen Y, Siegel F, Kipschull S, Haas B, Fröhlich H, Meister G, et al. miR-155 regulates differentiation of brown and beige adipocytes via a bistable circuit. Nat Commun. 2013;4(1):1–13.
Giroud M, Karbiener M, Pisani DF, Ghandour RA, Beranger GE, Niemi T, et al. Let-7i-5p represses brite adipocyte function in mice and humans. Sci Rep. 2016;6(1):1–11.
Giroud M, Pisani DF, Karbiener M, Barquissau V, Ghandour RA, Tews D, et al. miR-125b affects mitochondrial biogenesis and impairs brite adipocyte formation and function. Mol Metab. 2016;5(8):615.
West KA, Lagos D. Long non-coding RNA function in CD4+ T cells: what we know and what next? Non-Coding RNA. 2019;5(3):43.
De Jesus BB, Marinho SP, Arros S, Sousa-Franco A, Alves-Vale C, Carvalho T, et al. Silencing of the lncRNA Zeb2-NAT facilitates reprogramming of aged fibroblasts and safeguards stem cell pluripotency. Nat Commun. 2018;9(1):1–11.
Faghihi MA, Zhang M, Huang J, Modarresi F, Van der Brug MP, Nalls MA, et al. Evidence for natural antisense transcript-mediated inhibition of microRNA function. Genome Biol. 2010;11(5):1–13.
Zhang A, Zhao JC, Kim J, Fong KW, Yang YA, Chakravarti D, et al. LncRNA HOTAIR enhances the androgen-receptor-mediated transcriptional program and drives castration-resistant prostate cancer. Cell Rep. 2015;13(1):209–21.
Hu WL, Jin L, Xu A, Wang YF, Thorne RF, Zhang XD, et al. GUARDIN is a p53-responsive long non-coding RNA that is essential for genomic stability. Nat Cell Biol. 2018;20(4):492–502.
Lee S, Kopp F, Chang TC, Sataluri A, Chen B, Sivakumar S, et al. Noncoding RNA NORAD regulates genomic stability by sequestering PUMILIO proteins. Cell. 2016;164(1–2):69–80.
Shapira SN, Lim HW, Rajakumari S, Sakers AP, Ishibashi J, Harms MJ, et al. EBF2 transcriptionally regulates brown adipogenesis via the histone reader DPF3 and the BAF chromatin remodeling complex. Genes Dev. 2017;31(7):660–73.
Li S, Mi L, Yu L, Yu Q, Liu T, Wang GX, et al. Zbtb7b engages the long noncoding RNA Blnc1 to drive brown and beige fat development and thermogenesis. Proc Natl Acad Sci USA. 2017;114(34):E7111–20.
Clapier CR, Iwasa J, Cairns BR, Peterson CL. Mechanisms of action and regulation of ATP-dependent chromatin-remodelling complexes. Nat Rev Mol Cell Biol. 2017;18(7):407–22.
Puigserver P, Adelmant G, Wu Z, Fan M, Xu J, O’Malley B, et al. Activation of PPARγ coactivator-1 through transcription factor docking. Science (80-). 1999;286(5443):1368–71.
Roh HC, Tsai LTY, Lyubetskaya A, Tenen D, Kumari M, Rosen ED. Simultaneous transcriptional and epigenomic profiling from specific cell types within heterogeneous tissues in vivo. Cell Rep. 2017;18(4):1048–61.
Liu PS, Lin YW, Burton FH, Wei LN. Injecting engineered anti-inflammatory macrophages therapeutically induces white adipose tissue browning and improves diet-induced insulin resistance. Adipocyte. 2015;4(2):123–8.
Yang JP, Anderson AE, McCartney A, Ory X, Ma G, Pappalardo E, et al. Metabolically active three-dimensional brown adipose tissue engineered from white adipose-derived stem cells. Tissue Eng Part A. 2017;23(7–8):253–62.
Blumenfeld NR, Kang HJ, Fenzl A, Song Z, Chung JJ, Singh R, et al. A direct tissue-grafting approach to increasing endogenous brown fat. Sci Rep. 2018;8(1):7957.
Zhang Y, Liu Q, Yu J, Yu S, Wang J, Qiang L, et al. Locally induced adipose tissue browning by microneedle patch for obesity treatment. ACS Nano. 2017;11(9):9223–30.
Kir S, Komaba H, Garcia AP, Economopoulos KP, Liu W, Lanske B, et al. PTH/PTHrP receptor mediates cachexia in models of kidney failure and cancer. Cell Metab. 2016;23(2):315–23.
Auger C, Knuth CM, Abdullahi A, Samadi O, Parousis A, Jeschke MG. Metformin prevents the pathological browning of subcutaneous white adipose tissue. Mol Metab. 2019;29(August):12–23.
Balaz M, Becker AS, Balazova L, Straub L, Müller J, Gashi G, et al. Inhibition of mevalonate pathway prevents adipocyte browning in mice and men by affecting protein prenylation. Cell Metab. 2019;29(4):901-916.e8.
Lee P, Swarbrick MM, Ho KKY. Brown adipose tissue in adult humans: a metabolic renaissance. Endocr Rev. 2013;34(3):413–38.
Sharp LZ, Shinoda K, Ohno H, Scheel DW, Tomoda E, Ruiz L, et al. Human BAT possesses molecular signatures that resemble beige/brite cells. PLoS ONE. 2012;7(11):e49452.
Mo Q, Salley J, Roshan T, Baer LA, May FJ, Jaehnig EJ, et al. Identification and characterization of a supraclavicular brown adipose tissue in mice. JCI Insight. 2017;2(11):e93166.
de Jong JMA, Sun W, Pires ND, Frontini A, Balaz M, Jespersen NZ, et al. Human brown adipose tissue is phenocopied by classical brown adipose tissue in physiologically humanized mice. Nat Metab. 2019;1(8):830–43.
Kajimura S, Spiegelman BM. Confounding issues in the ‘humanized’ BAT of mice. Nat Metab. 2020;2(4):303–4.
de Jong JMA, Cannon B, Nedergaard J, Wolfrum C, Petrovic N. Reply to ‘confounding issues in the “humanized” brown fat of mice.’ Nat Metab. 2020;2(4):305–6.
Download references
Author information
Sabrina Azevedo Machado and Gabriel Pasquarelli-do-Nascimento have contributed equally to this work.
Authors and Affiliations
Laboratory of Immunology and Inflammation, Department of Cell Biology, University of Brasilia, Brasília, DF, Brazil
Sabrina Azevedo Machado, Gabriel Pasquarelli-do-Nascimento, Debora Santos da Silva, Gabriel Ribeiro Farias, Igor de Oliveira Santos, Luana Borges Baptista & Kelly Grace Magalhães
You can also search for this author in PubMed Google Scholar
Contributions
SAM, GP, GFR, LBB, DSS, and IOS wrote different sections of the manuscript. IOS prepared the figure. KGM prepared, revised, and wrote the manuscript. All authors listed have made a substantial, direct, and intellectual contribution to the work and approved it for publication. All authors read and approved the final manuscript.
Corresponding author
Correspondence to Kelly Grace Magalhães .
Ethics declarations
Ethics approval and consent to participate, consent for publication, competing interests.
The authors declare that they have no competing interests.
Additional information
Publisher's note.
Springer Nature remains neutral with regard to jurisdictional claims in published maps and institutional affiliations.
Rights and permissions
Open Access This article is licensed under a Creative Commons Attribution 4.0 International License, which permits use, sharing, adaptation, distribution and reproduction in any medium or format, as long as you give appropriate credit to the original author(s) and the source, provide a link to the Creative Commons licence, and indicate if changes were made. The images or other third party material in this article are included in the article's Creative Commons licence, unless indicated otherwise in a credit line to the material. If material is not included in the article's Creative Commons licence and your intended use is not permitted by statutory regulation or exceeds the permitted use, you will need to obtain permission directly from the copyright holder. To view a copy of this licence, visit http://creativecommons.org/licenses/by/4.0/ . The Creative Commons Public Domain Dedication waiver ( http://creativecommons.org/publicdomain/zero/1.0/ ) applies to the data made available in this article, unless otherwise stated in a credit line to the data.
Reprints and permissions
About this article
Cite this article.
Machado, S.A., Pasquarelli-do-Nascimento, G., da Silva, D. et al. Browning of the white adipose tissue regulation: new insights into nutritional and metabolic relevance in health and diseases. Nutr Metab (Lond) 19 , 61 (2022). https://doi.org/10.1186/s12986-022-00694-0
Download citation
Received : 19 May 2022
Accepted : 19 August 2022
Published : 06 September 2022
DOI : https://doi.org/10.1186/s12986-022-00694-0
Share this article
Anyone you share the following link with will be able to read this content:
Sorry, a shareable link is not currently available for this article.
Provided by the Springer Nature SharedIt content-sharing initiative
Nutrition & Metabolism
ISSN: 1743-7075
- General enquiries: [email protected]
Thank you for visiting nature.com. You are using a browser version with limited support for CSS. To obtain the best experience, we recommend you use a more up to date browser (or turn off compatibility mode in Internet Explorer). In the meantime, to ensure continued support, we are displaying the site without styles and JavaScript.
- View all journals
- My Account Login
- Explore content
- About the journal
- Publish with us
- Sign up for alerts
- Open access
- Published: 08 April 2024
Large-scale phenotyping of patients with long COVID post-hospitalization reveals mechanistic subtypes of disease
- Felicity Liew 1 na1 ,
- Claudia Efstathiou ORCID: orcid.org/0000-0001-6125-8126 1 na1 ,
- Sara Fontanella 1 ,
- Matthew Richardson 2 ,
- Ruth Saunders 2 ,
- Dawid Swieboda 1 ,
- Jasmin K. Sidhu 1 ,
- Stephanie Ascough 1 ,
- Shona C. Moore ORCID: orcid.org/0000-0001-8610-2806 3 ,
- Noura Mohamed 4 ,
- Jose Nunag ORCID: orcid.org/0000-0002-4218-0500 5 ,
- Clara King 5 ,
- Olivia C. Leavy 2 , 6 ,
- Omer Elneima 2 ,
- Hamish J. C. McAuley 2 ,
- Aarti Shikotra 7 ,
- Amisha Singapuri ORCID: orcid.org/0009-0002-4711-7516 2 ,
- Marco Sereno ORCID: orcid.org/0000-0003-4573-9303 2 ,
- Victoria C. Harris 2 ,
- Linzy Houchen-Wolloff ORCID: orcid.org/0000-0003-4940-8835 8 ,
- Neil J. Greening ORCID: orcid.org/0000-0003-0453-7529 2 ,
- Nazir I. Lone ORCID: orcid.org/0000-0003-2707-2779 9 ,
- Matthew Thorpe 10 ,
- A. A. Roger Thompson ORCID: orcid.org/0000-0002-0717-4551 11 ,
- Sarah L. Rowland-Jones 11 ,
- Annemarie B. Docherty ORCID: orcid.org/0000-0001-8277-420X 10 ,
- James D. Chalmers 12 ,
- Ling-Pei Ho ORCID: orcid.org/0000-0001-8319-301X 13 ,
- Alexander Horsley ORCID: orcid.org/0000-0003-1828-0058 14 ,
- Betty Raman 15 ,
- Krisnah Poinasamy 16 ,
- Michael Marks 17 , 18 , 19 ,
- Onn Min Kon 1 ,
- Luke S. Howard ORCID: orcid.org/0000-0003-2822-210X 1 ,
- Daniel G. Wootton 3 ,
- Jennifer K. Quint 1 ,
- Thushan I. de Silva ORCID: orcid.org/0000-0002-6498-9212 11 ,
- Antonia Ho 20 ,
- Christopher Chiu ORCID: orcid.org/0000-0003-0914-920X 1 ,
- Ewen M. Harrison ORCID: orcid.org/0000-0002-5018-3066 10 ,
- William Greenhalf 21 ,
- J. Kenneth Baillie ORCID: orcid.org/0000-0001-5258-793X 10 , 22 , 23 ,
- Malcolm G. Semple ORCID: orcid.org/0000-0001-9700-0418 3 , 24 ,
- Lance Turtle 3 , 24 ,
- Rachael A. Evans ORCID: orcid.org/0000-0002-1667-868X 2 ,
- Louise V. Wain 2 , 6 ,
- Christopher Brightling 2 ,
- Ryan S. Thwaites ORCID: orcid.org/0000-0003-3052-2793 1 na1 ,
- Peter J. M. Openshaw ORCID: orcid.org/0000-0002-7220-2555 1 na1 ,
- PHOSP-COVID collaborative group &
ISARIC investigators
Nature Immunology volume 25 , pages 607–621 ( 2024 ) Cite this article
18k Accesses
1 Citations
2017 Altmetric
Metrics details
- Inflammasome
- Inflammation
- Innate immunity
One in ten severe acute respiratory syndrome coronavirus 2 infections result in prolonged symptoms termed long coronavirus disease (COVID), yet disease phenotypes and mechanisms are poorly understood 1 . Here we profiled 368 plasma proteins in 657 participants ≥3 months following hospitalization. Of these, 426 had at least one long COVID symptom and 233 had fully recovered. Elevated markers of myeloid inflammation and complement activation were associated with long COVID. IL-1R2, MATN2 and COLEC12 were associated with cardiorespiratory symptoms, fatigue and anxiety/depression; MATN2, CSF3 and C1QA were elevated in gastrointestinal symptoms and C1QA was elevated in cognitive impairment. Additional markers of alterations in nerve tissue repair (SPON-1 and NFASC) were elevated in those with cognitive impairment and SCG3, suggestive of brain–gut axis disturbance, was elevated in gastrointestinal symptoms. Severe acute respiratory syndrome coronavirus 2-specific immunoglobulin G (IgG) was persistently elevated in some individuals with long COVID, but virus was not detected in sputum. Analysis of inflammatory markers in nasal fluids showed no association with symptoms. Our study aimed to understand inflammatory processes that underlie long COVID and was not designed for biomarker discovery. Our findings suggest that specific inflammatory pathways related to tissue damage are implicated in subtypes of long COVID, which might be targeted in future therapeutic trials.
Similar content being viewed by others
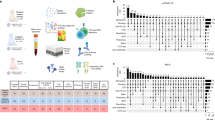
Immunopathological signatures in multisystem inflammatory syndrome in children and pediatric COVID-19
Keith Sacco, Riccardo Castagnoli, … Luigi D. Notarangelo
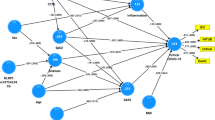
In COVID-19, NLRP3 inflammasome genetic variants are associated with critical disease and these effects are partly mediated by the sickness symptom complex: a nomothetic network approach
Michael Maes, Walton Luiz Del Tedesco Junior, … Andréa Name Colado Simão
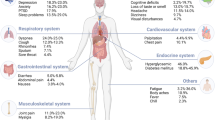
Epidemiology, clinical presentation, pathophysiology, and management of long COVID: an update
Sizhen Su, Yimiao Zhao, … Lin Lu
One in ten severe acute respiratory syndrome coronavirus 2 (SARS-CoV-2) infections results in post-acute sequelae of coronavirus disease 2019 (PASC) or long coronavirus disease (COVID), which affects 65 million people worldwide 1 . Long COVID (LC) remains common, even after mild acute infection with recent variants 2 , and it is likely LC will continue to cause substantial long-term ill health, requiring targeted management based on an understanding of how disease phenotypes relate to underlying mechanisms. Persistent inflammation has been reported in adults with LC 1 , 3 , but studies have been limited in size, timing of samples or breadth of immune mediators measured, leading to inconsistent or absent associations with symptoms. Markers of oxidative stress, metabolic disturbance, vasculoproliferative processes and IFN-, NF-κB- or monocyte-related inflammation have been suggested 3 , 4 , 5 , 6 .
The PHOSP-COVID study, a multicenter United Kingdom study of patients previously hospitalized with COVID-19, has reported inflammatory profiles in 626 adults with health impairment after COVID-19, identified through clustering. Elevated IL-6 and markers of mucosal inflammation were observed in those with severe impairment compared with individuals with milder impairment 7 . However, LC is a heterogeneous condition that may be a distinct form of health impairment after COVID-19, and it remains unclear whether there are inflammatory changes specific to LC symptom subtypes. Determining whether activated inflammatory pathways underlie all cases of LC or if mechanisms differ according to clinical presentation is essential for developing effective therapies and has been highlighted as a top research priority by patients and clinicians 8 .
In this Letter, in a prospective multicenter study, we measured 368 plasma proteins in 657 adults previously hospitalized for COVID-19 (Fig. 1a and Table 1 ). Individuals in our cohort experienced a range of acute COVID-19 severities based on World Health Organization (WHO) progression scores 9 ; WHO 3–4 (no oxygen support, n = 133 and median age of 55 years), WHO 5–6 (oxygen support, n = 353 and median age of 59 years) and WHO 7–9 (critical care, n = 171 and median age of 57 years). Participants were hospitalized for COVID-19 ≥3 months before sample collection (median 6.1 months, interquartile range (IQR) 5.1–6.8 months and range 3.0–8.3 months) and confirmed clinically ( n = 36/657) or by PCR ( n = 621/657). Symptom data indicated 233/657 (35%) felt fully recovered at 6 months (hereafter ‘recovered’) and the remaining 424 (65%) reported symptoms consistent with the WHO definition for LC (symptoms ≥3 months post infection 10 ). Given the diversity of LC presentations, patients were grouped according to symptom type (Fig. 1b ). Groups were defined using symptoms and health deficits that have been commonly reported in the literature 1 ( Methods ). A multivariate penalized logistic regression model (PLR) was used to explore associations of clinical covariates and immune mediators at 6 months between recovered patients ( n = 233) and each LC group (cardiorespiratory symptoms, cardioresp, n = 398, Fig. 1c ; fatigue, n = 384, Fig. 1d ; affective symptoms, anxiety/depression, n = 202, Fig. 1e ; gastrointestinal symptoms, GI, n = 132, Fig. 1f ; and cognitive impairment, cognitive, n = 61, Fig. 1g ). Women ( n = 239) were more likely to experience CardioResp (odds ratio (OR 1.14), Fatigue (OR 1.22), GI (OR 1.13) and Cognitive (OR 1.03) outcomes (Fig. 1c,d,f,g ). Repeated cross-validation was used to optimize and assess model performance ( Methods and Extended Data Fig. 1 ). Pre-existing conditions, such as chronic lung disease, neurological disease and cardiovascular disease (Supplementary Table 1 ), were associated with all LC groups (Fig. 1c–g ). Age, C-reactive protein (CRP) and acute disease severity were not associated with any LC group (Table 1 ).
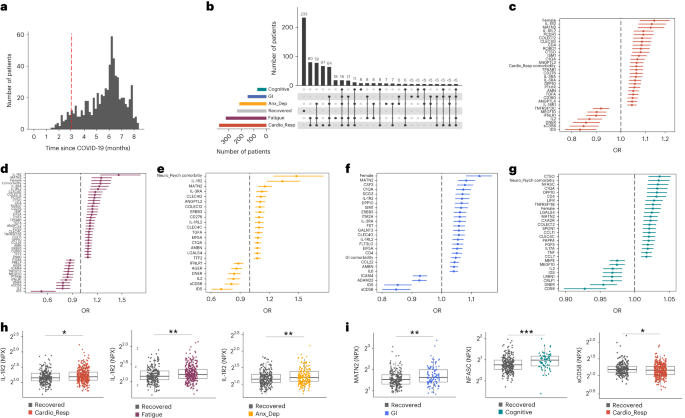
a , Distribution of time from COVID-19 hospitalization at sample collection. All samples were cross-sectional. The vertical red line indicates the 3 month cutoff used to define our final cohort and samples collected before 3 months were excluded. b , An UpSet plot describing pooled LC groups. The horizontal colored bars represent the number of patients in each symptom group: cardiorespiratory (Cardio_Resp), fatigue, cognitive, GI and anxiety/depression (Anx_Dep). Vertical black bars represent the number of patients in each symptom combination group. To prevent patient identification, where less than five patients belong to a combination group, this has been represented as ‘<5’. The recovered group ( n = 233) were used as controls. c – g , Forest plots of Olink protein concentrations (NPX) associated with Cardio_Resp ( n = 365) ( c ), fatigue (n = 314) ( d ), Anx_Dep ( n = 202) ( e ), GI ( n = 124) ( f ) and cognitive ( n = 60) ( g ). Neuro_Psych, neuropsychiatric. The error bars represent the median accuracy of the model. h , i , Distribution of Olink values (NPX) for IL-1R2 ( h ) and MATN2, neurofascin and sCD58 ( i ) measured between symptomatic and recovered individuals in recovered ( n = 233), Cardio_Resp ( n = 365), fatigue ( n = 314) and Anx_Dep ( n = 202) groups ( h ) and MATN2 in GI ( n = 124), neurofascin in cognitive ( n = 60) and sCD58 in Cardio_Resp and recovered groups ( i ). The box plot center line represents the median, the boundaries represent IQR and the whisker length represents 1.5× IQR. The median values were compared between groups using two-sided Wilcoxon signed-rank test, * P < 0.05, ** P < 0.01, *** P < 0.001 and **** P < 0.0001.
To study the association of peripheral inflammation with symptoms, we analyzed cross-sectional data collected approximately 6 months after hospitalizations. We measured 368 immune mediators from plasma collected contemporaneously with symptom data. Mediators suggestive of myeloid inflammation were associated with all symptoms (Fig. 1c–h ). Elevated IL-1R2, an IL-1 receptor expressed by monocytes and macrophages modulating inflammation 11 and MATN2, an extracellular matrix protein that modulates tissue inflammation through recruitment of innate immune cells 12 , were associated with cardioresp (IL-1R2 OR 1.14, Fig. 1c,h ), fatigue (IL-1R2 OR 1.45, Fig. 1d,h ), anxiety/depression (IL-1R2 OR 1.34. Fig. 1e,h ) and GI (MATN2 OR 1.08, Fig. 1f ). IL-3RA, an IL-3 receptor, was associated with cardioresp (OR 1.07, Fig. 1c ), fatigue (OR 1.21, Fig. 1d ), anxiety/depression (OR 1.12, Fig. 1e ) and GI (OR 1.06, Fig. 1f ) groups, while CSF3, a cytokine promoting neutrophilic inflammation 13 , was elevated in cardioresp (OR 1.06, Fig. 1c ), fatigue (OR 1.12, Fig. 1d ) and GI (OR 1.08, Fig. 1f ).
Elevated COLEC12, which initiates inflammation in tissues by activating the alternative complement pathway 14 , associated with cardioresp (OR 1.09, Fig. 1c ), fatigue (OR 1.19, Fig. 1d ) and anxiety/depression (OR 1.11, Fig. 1e ), but not with GI (Fig. 1f ) and only weakly with cognitive (OR 1.02, Fig. 1g ). C1QA, a degradation product released by complement activation 15 was associated with GI (OR 1.08, Fig. 1f ) and cognitive (OR 1.03, Fig. 1g ). C1QA, which is known to mediate dementia-related neuroinflammation 16 , had the third strongest association with cognitive (Fig. 1g ). These observations indicated that myeloid inflammation and complement activation were associated with LC.
Increased expression of DPP10 and SCG3 was observed in the GI group compared with recovered (DPP10 OR 1.07 and SCG3 OR 1.08, Fig. 1f ). DPP10 is a membrane protein that modulates tissue inflammation, and increased DPP10 expression is associated with inflammatory bowel disease 17 , 18 , suggesting that GI symptoms may result from enteric inflammation. Elevated SCG3, a multifunctional protein that has been associated with irritable bowel syndrome 19 , suggested that noninflammatory disturbance of the brain–gut axis or dysbiosis, may occur in the GI group. The cognitive group was associated with elevated CTSO (OR 1.04), NFASC (OR 1.03) and SPON-1 (OR 1.02, Fig. 1g,i ). NFASC and SPON-1 regulate neural growth 20 , 21 , while CTSO is a cysteine proteinase supporting tissue turnover 22 . The increased expression of these three proteins as well as C1QA and DPP10 in the cognitive group (Fig. 1g ) suggested neuroinflammation and alterations in nerve tissue repair, possibly resulting in neurodegeneration. Together, our findings indicated that complement activation and myeloid inflammation were common to all LC groups, but subtle differences were observed in the GI and cognitive groups, which may have mechanistic importance. Acutely elevated fibrinogen during hospitalization has been reported to be predictive of LC cognitive deficits 23 . We found elevated fibrinogen in LC relative to recovered (Extended Data Fig. 2a ; P = 0.0077), although this was not significant when restricted to the cognitive group ( P = 0.074), supporting our observation of complement pathway activation in LC and in keeping with reports that complement dysregulation and thrombosis drive severe COVID-19 (ref. 24 ).
Elevated sCD58 was associated with lower odds of all LC symptoms and was most pronounced in cardioresp (OR 0.85, Fig. 1c,i ), fatigue (OR 0.80, Fig. 1d ) and anxiety/depression (OR 0.83, Fig. 1e ). IL-2 was negatively associated with the cardioresp (Fig. 1c , OR 0.87), fatigue (Fig. 1d , OR 0.80), anxiety/depression (Fig. 1e , OR 0.84) and cognitive (Fig. 1g , OR 0.96) groups. Both IL-2 and sCD58 have immunoregulatory functions 25 , 26 . Specifically, sCD58 suppresses IL-1- or IL-6-dependent interactions between CD2 + monocytes and CD58 + T or natural killer cells 26 . The association of sCD58 with recovered suggests a central role of dysregulated myeloid inflammation in LC. Elevated markers of tissue repair, IDS and DNER 27 , 28 , were also associated with recovered relative to all LC groups (Fig. 1c–g ). Taken together, our data suggest that suppression of myeloid inflammation and enhanced tissue repair were associated with recovered, supporting the use of immunomodulatory agents in therapeutic trials 29 (Supplementary Table 2 ).
We next sought to validate the experimental and analytical approaches used. Although Olink has been validated against other immunoassay platforms, showing superior sensitivity and specificity 30 , 31 , we confirmed the performance of Olink against chemiluminescent immunoassays within our cohort. We performed chemiluminescent immunoassays on plasma from a subgroup of 58 participants (recovered n = 13 and LC n = 45). There were good correlations between results from Olink (normalized protein expression (NPX)) and chemiluminescent immunoassays (pg ml −1 ) for CSF3, IL-1R2, IL-3RA, TNF and TFF2 (Extended Data Fig. 3 ). Most samples did not have concentrations of IL-2 detectable using a mesoscale discovery chemiluminescent assay, limiting this analysis to 14 samples (recovered n = 4, LC n = 10, R = 0.55 and P = 0.053, Extended Data Fig. 3 ). We next repeated our analysis using alternative definitions of LC. The Centers for Disease Control and Prevention and National Institute for Health and Care Excellence definitions for LC include symptoms occurring 1 month post infection 32 , 33 . Using the 1 month post-infection definition included 62 additional participants to our analysis (recovered n = 21, 3 females and median age 61 years and LC n = 41, 15 females and median age 60 years, Extended Data Fig. 2c ) and found that inflammatory associations with each LC group were consistent with our analysis based on the WHO definition (Extended Data Fig. 2d–h ). Finally, to validate the analytical approach (PLR) we examined the distribution of data, prioritizing proteins that were most strongly associated with each LC/recovered group (IL-1R2, MATN2, NFASC and sCD58). Each protein was significantly elevated in the LC group compared with recovered (Fig. 1h,i and Extended Data Fig. 4 ), consistent with the PLR. Alternative regression approaches (unadjusted regression models and partial least squares, PLS) reported results consistent with the original analysis of protein associations and LC outcome in the WHO-defined cohort (Fig. 1c–g , Supplementary Table 3 and Extended Data Figs. 5 and 6 ). The standard errors of PLS estimates were wide (Extended Data Fig. 6 ), consistent with previous demonstrations that PLR is the optimal method to analyze high-dimensional data where variables may have combined effects 34 . As inflammatory proteins are often colinear, working in-tandem to mediate effects, we prioritized PLR results to draw conclusions.
To explore the relationship between inflammatory mediators associated with different LC symptoms, we performed a network analysis of Olink mediators highlighted by PLR within each LC group. COLEC12 and markers of endothelial and mucosal inflammation (MATN2, PCDH1, ROBO1, ISM1, ANGPTL2, TGF-α and TFF2) were highly correlated within the cardioresp, fatigue and anxiety/depression groups (Fig. 2 and Extended Data Fig. 7 ). Elevated PCDH1, an adhesion protein modulating airway inflammation 35 , was highly correlated with other inflammatory proteins associated with the cardioresp group (Fig. 2 ), suggesting that systemic inflammation may arise from the lung in these individuals. This was supported by increased expression of IL-3RA, which regulates innate immune responses in the lung through interactions with circulating IL-3 (ref. 36 ), in fatigue (Figs. 1d and 2 ), which correlated with markers of tissue inflammation, including PCDH1 (Fig. 2 ). MATN2 and ISM1, mucosal proteins that enhance inflammation 37 , 38 , were highly correlated in the GI group (Fig. 2 ), highlighting the role of tissue-specific inflammation in different LC groups. SCG3 correlated less closely with mediators in the GI group (Fig. 2 ), suggesting that the brain–gut axis may contribute separately to some GI symptoms. SPON-1, which regulates neural growth 21 , was the most highly correlated mediator in the cognitive group (Fig. 2 and Extended Data Fig. 7 ), highlighting that processes within nerve tissue may underlie this group. These observations suggested that inflammation might arise from mucosal tissues and that additional mechanisms may contribute to pathophysiology underlying the GI and cognitive groups.
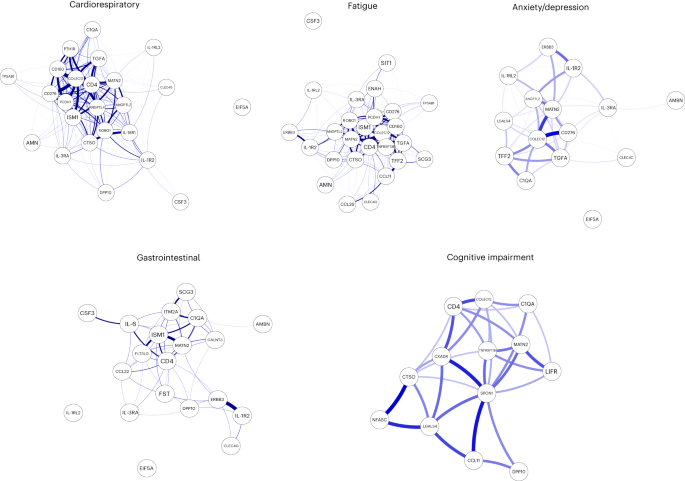
Network analysis of Olink mediators associated with cardioresp ( n = 365), fatigue ( n = 314), anxiety/depression ( n = 202), GI ( n = 124) and cognitive groups ( n = 60). Each node corresponds to a protein mediator identified by PLR. The edges (blue lines) were weighted according to the size of Spearman’s rank correlation coefficient between proteins. All edges represent positive and significant correlations ( P < 0.05) after FDR adjustment.
Women were more likely to experience LC (Table 1 ), as found in previous studies 1 . As estrogen can influence immunological responses 39 , we investigated whether hormonal differences between men and women with LC in our cohort explained this trend. We grouped men and women with LC symptoms into two age groups (those younger than 50 years and those 50 years and older, using age as a proxy for menopause status in women) and compared mediator levels between men and women in each age group, prioritizing those identified by PLR to be higher in LC compared with recovered. As we aimed to understand whether women with LC had stronger inflammatory responses than men with LC, we did not assess differences in men and women in the recovered group. IL-1R2 and MATN2 were significantly higher in women ≥50 years than men ≥50 years in the cardioresp group (Fig. 3a , IL-1R2 and MATN2) and the fatigue group (Fig. 3b ). In the GI group, CSF3 was higher in women ≥50 years compared with men ≥50 years (Fig. 3c ), indicating that the inflammatory markers observed in women were not likely to be estrogen-dependent. Women have been reported to have stronger innate immune responses to infection and to be at greater risk of autoimmunity 39 , possibly explaining why some women in the ≥50 years group had higher inflammatory proteins than men the same group. Proteins associated with the anxiety/depression (IL-1R2 P = 0.11 and MATN2 P = 0.61, Extended Data Fig. 8a ) and cognitive groups (CTSO P = 0.64 and NFASC P = 0.41, Extended Data Fig. 8b ) were not different between men and women in either age group, consistent with the absent/weak association between sex and these outcomes identified by PLR (Fig. 1e,g ). Though our findings suggested that nonhormonal differences in inflammatory responses may explain why some women are more likely to have LC, they require confirmation in adequately powered studies.

a – c , Olink-measured plasma protein levels (NPX) of IL-1R2 and MATN2 ( a and b ) and CSF3 ( c ) between LC men and LC women divided by age (<50 or ≥50 years) in the cardiorespiratory group (<50 years n = 8 and ≥50 years n = 270) ( a ), fatigue group (<50 years n = 81 and ≥50 years n = 227) ( b ) and GI group (<50 years n = 34 and ≥50 years n = 82) ( c ). the median values were compared between men and women using two-sided Wilcoxon signed-rank test, * P < 0.05, ** P < 0.01, *** P < 0.001 and **** P < 0.0001. The box plot center line represents the median, the boundaries represent IQR and the whisker length represents 1.5× IQR.
To test whether local respiratory tract inflammation persisted after COVID-19, we compared nasosorption samples from 89 participants (recovered, n = 31; LC, n = 33; and healthy SARS-CoV-2 naive controls, n = 25, Supplementary Tables 4 and 5 ). Several inflammatory markers were elevated in the upper respiratory tract post COVID (including IL-1α, CXCL10, CXCL11, TNF, VEGF and TFF2) when compared with naive controls, but similar between recovered and LC (Fig. 4a ). In the cardioresp group ( n = 29), inflammatory mediators elevated in plasma (for example, IL-6, APO-2, TGF-α and TFF2) were not elevated in the upper respiratory tract (Extended Data Fig. 9a ) and there was no correlation between plasma and nasal mediator levels (Extended Data Fig. 9b ). This exploratory analysis suggested upper respiratory tract inflammation post COVID was not specifically associated with cardiorespiratory symptoms.
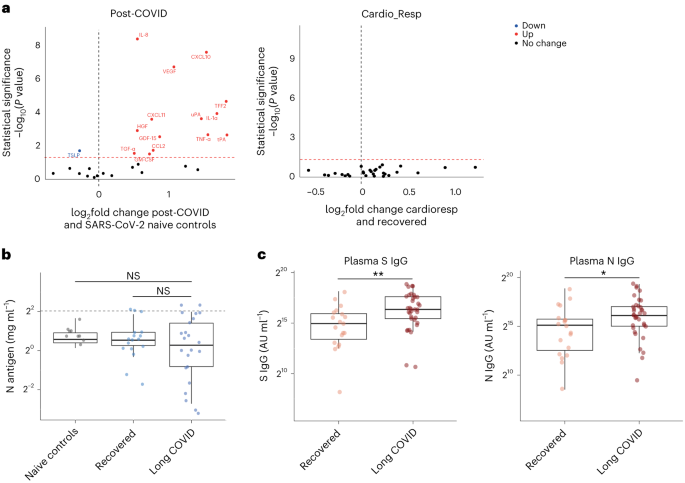
a , Nasal cytokines measured by immunoassay in post-COVID participants ( n = 64) compared with healthy SARS-CoV-2 naive controls ( n = 25), and between the the cardioresp group ( n = 29) and the recovered group ( n = 31). The red values indicate significantly increased cytokine levels after FDR adjustment ( P < 0.05) using two-tailed Wilcoxon signed-rank test. b , SARS-CoV-2 N antigen measured in sputum by electrochemiluminescence from recovered ( n = 17) and pooled LC ( n = 23) groups, compared with BALF from SARS-CoV-2 naive controls ( n = 9). The horizontal dashed line indicates the lower limit of detection of the assay. c , Plasma S- and N-specific IgG responses measured by electrochemiluminescence in the LC ( n = 35) and recovered ( n = 19) groups. The median values were compared using two-sided Wilcoxon signed-rank tests, NS P > 0.05, * P < 0.05, ** P < 0.01, *** P < 0.001 and **** P < 0.0001. The box plot center lines represent the median, the boundaries represent IQR and the whisker length represents 1.5× IQR.
To explore whether SARS-CoV-2 persistence might explain the inflammatory profiles observed in the cardioresp group, we measured SARS-CoV-2 nucleocapsid (N) antigen in sputum from 40 participants (recovered n = 17 and LC n = 23) collected approximately 6 months post hospitalization (Supplementary Table 6 ). All samples were compared with prepandemic bronchoalveolar lavage fluid ( n = 9, Supplementary Table 4 ). Only four samples (recovered n = 2 and LC n = 2) had N antigen above the assay’s lower limit of detection, and there was no difference in N antigen concentrations between LC and recovered (Fig. 4b , P = 0.78). These observations did not exclude viral persistence, which might require tissues samples for detection 40 , 41 . On the basis of the hypothesis that persistent viral antigen might prevent a decline in antibody levels over time, we examined the titers of SARS-CoV-2-specific antibodies in unvaccinated individuals (recovered n = 19 and LC n = 35). SARS-CoV-2 N-specific ( P = 0.023) and spike (S)-specific ( P = 0.0040) immunoglobulin G (IgG) levels were elevated in LC compared with recovered (Fig. 4c ).
Overall, we identified myeloid inflammation and complement activation in the cardioresp, fatigue, anxiety/depression, cognitive and GI groups 6 months after hospitalization (Extended Data Fig. 10 ). Our findings build on results of smaller studies 5 , 6 , 42 and are consistent with a genome-wide association study that identified an independent association between LC and FOXP4 , which modulates neutrophilic inflammation and immune cell function 43 , 44 . In addition, we identified tissue-specific inflammatory elements, indicating that myeloid disturbance in different tissues may result in distinct symptoms. Multiple mechanisms for LC have been suggested, including autoimmunity, thrombosis, vascular dysfunction, SARS-CoV-2 persistence and latent virus reactivation 1 . All these processes involve myeloid inflammation and complement activation 45 . Complement activation in LC has been suggested in a proteomic study in 97 mostly nonhospitalized COVID-19 cases 42 and a study of 48 LC patients, of which one-third experienced severe acute disease 46 . As components of the complement system are known to have a short half-life 47 , ongoing complement activation suggests active inflammation rather than past tissue damage from acute infection.
Despite the heterogeneity of LC and the likelihood of coexisting or multiple etiologies, our work suggests some common pathways that might be targeted therapeutically and supports the rationale for several drugs currently under trial. Our finding of increased sCD58 levels (associated with suppression of monocyte–lymphocyte interactions 26 ) in the recovered group, strengthens our conclusion that myeloid inflammation is central to the biology of LC and that trials of steroids, IL-1 antagonists, JAK inhibitors, naltrexone and colchicine are justified. Although anticoagulants such as apixaban might prevent thrombosis downstream of complement dysregulation, they can also increase the risk of serious bleeding when given after COVID-19 hospitalization 48 . Thus, clinical trials, already underway, need to carefully assess the risks and benefits of anticoagulants (Supplementary Table 2 ).
Our finding of elevated S- and N-specific IgG in LC could suggest viral persistence, as found in other studies 6 , 42 , 49 . Our network analysis indicated that inflammatory proteins in the cardioresp group interacted strongly with ISM1 and ROBO1, which are expressed during respiratory tract infection and regulate lung inflammation 50 , 51 . Although we were unable to find SARS-CoV-2 antigen in sputum from our LC cases, we did not test for viral persistence in GI tract and lung tissue 40 , 41 or in plasma 52 . Evidence of SARS-CoV-2 persistence would justify trials of antiviral drugs (singly or in combination) in LC. It is also possible that autoimmune processes could result in an innate inflammatory profile in LC. Autoreactive B cells have been identified in LC patients with higher SARS-CoV-2-specific antibody titers in a study of mostly mild acute COVID cases (59% WHO 2–3) 42 , a different population from our study of hospitalized cases.
Our observations of distinct protein profiles in GI and cognitive groups support previous reports on distinct associations between Epstein–Barr virus reactivation and neurological symptoms, or autoantibodies and GI symptoms relative to other forms of LC 49 , 53 . We did not assess autoantibody induction but found evidence of brain–gut axis disturbance (SCG3) in the GI group, which occurs in many autoimmune diseases 54 . We found signatures suggestive of neuroinflammation (C1QA) in the cognitive group, consistent with findings of brain abnormalities on magnetic resonance imaging after COVID-19 hospitalization 55 , as well as findings of microglial activation in mice after COVID-19 (ref. 56 ). Proinflammatory signatures dominated in the cardioresp, fatigue and anxiety/depression groups and were consistent with those seen in non-COVID depression, suggesting shared mechanisms 57 . The association between markers of myeloid inflammation, including IL-3RA, and symptoms was greatest for fatigue. Whilst membrane-bound IL-3RA facilitates IL-3 signaling upstream of myelopoesis 36 its soluble form (measured in plasma) can bind IL-3 and can act as a decoy receptor, preventing monocyte maturation and enhancing immunopathology 58 . Monocytes from individuals with post-COVID fatigue are reported to have abnormal expression profiles (including reduced CXCR2), suggestive of altered maturation and migration 5 , 59 . Lung-specific inflammation was suggested by the association between PCDH1 (an airway epithelial adhesion molecule 35 ) and cardioresp symptoms.
Our observations do not align with all published observations on LC. One proteomic study of 55 LC cases after generally mild (WHO 2–3) acute disease found that TNF and IFN signatures were elevated in LC 3 . Vasculoproliferative processes and metabolic disturbance have been reported in LC 4 , 60 , but these studies used uninfected healthy individuals for comparison and cannot distinguish between LC-specific phenomena and residual post-COVID inflammation. A study of 63 adults (LC, n = 50 and recovered, n = 13) reported no association between immune cell activation and LC 3 months after infection 61 , though myeloid inflammation was not directly measured, and 3 months post infection may be too early to detect subtle differences between LC and recovered cases due to residual acute inflammation.
Our study has limitations. We designed the study to identify inflammatory markers identifying pathways underlying LC subgroups rather than diagnostic biomarkers. The ORs we report are small, but associations were consistent across alternative methods of analysis and when using different LC definitions. Small effect sizes can be expected when using PLR, which shrinks correlated mediator coefficients to reflect combined effects and prevent colinear inflation 62 , and could also result from measurement of plasma mediators that may underestimate tissue inflammation. Although our LC cohort is large compared with most other published studies, some of our subgroups are small (only 60 cases were designated cognitive). Though the performance of the cognitive PLR model was adequate, our findings should be validated in larger studies. It should be noted that our cohort of hospitalized cases may not represent all types of LC, especially those occurring after mild infection. We looked for an effect of acute disease severity within our study and did not find it, and are reassured that the inflammatory profiles we observed were consistent with those seen in smaller studies including nonhospitalized cases 42 , 46 . Studies of posthospital LC may be confounded by ‘posthospital syndrome’, which encompasses general and nonspecific effects of hospitalization (particularly intensive care) 63 .
In conclusion, we found markers of myeloid inflammation and complement activation in our large prospective posthospital cohort of patients with LC, in addition to distinct inflammatory patterns in patients with cognitive impairment or gastrointestinal symptoms. These findings show the need to consider subphenotypes in managing patients with LC and support the use of antiviral or immunomodulatory agents in controlled therapeutic trials.
Study design and ethics
After hospitalization for COVID-19, adults who had no comorbidity resulting in a prognosis of less than 6 months were recruited to the PHOSP-COVID study ( n = 719). Patients hospitalized between February 2020 and January 2021 were recruited. Both sexes were recruited and gender was self-reported (female, n = 257 and male, n = 462). Written informed consent was obtained from all patients. Ethical approvals for the PHOSP-COVID study were given by Leeds West Research Ethics Committee (20/YH/0225).
Symptom data and samples were prospectively collected from individuals approximately 6 months (IQR 5.1–6.8 months and range 3.0–8.3 months) post hospitalization (Fig. 1a ), via the PHOSP-COVID multicenter United Kingdom study 64 . Data relating to patient demographics and acute admission were collected via the International Severe Acute Respiratory and Emerging Infection Consortium World Health Organization Clinical Characterisation Protocol United Kingdom (ISARIC4C study; IRAS260007/IRAS126600) (ref. 65 ). Adults hospitalized during the SARS-CoV-2 pandemic were systematically recruited into ISARIC4C. Written informed consent was obtained from all patients. Ethical approval was given by the South Central–Oxford C Research Ethics Committee in England (reference 13:/SC/0149), Scotland A Research Ethics Committee (20/SS/0028) and WHO Ethics Review Committee (RPC571 and RPC572l, 25 April 2013).
Data were collected to account for variables affecting symptom outcome, via hospital records and self-reporting. Acute disease severity was classified according to the WHO clinical progression score: WHO class 3–4: no oxygen therapy; class 5: oxygen therapy; class 6: noninvasive ventilation or high-flow nasal oxygen; and class 7–9: managed in critical care 9 . Clinical data were used to place patients into six categories: ‘recovered’, ‘GI’, ‘cardiorespiratory’, ‘fatigue’, ‘cognitive impairment’ and ‘anxiety/depression’ (Supplementary Table 7 ). Patient-reported symptoms and validated clinical scores were used when feasible, including Medical Research Council (MRC) breathlessness score, dyspnea-12 score, Functional Assessment of Chronic Illness Therapy (FACIT) score, Patient Health Questionnaire (PHQ)-9 and Generalized Anxiety Disorder (GAD)-7. Cognitive impairment was defined as a Montreal Cognitive Assessment score <26. GI symptoms were defined as answering ‘Yes’ to the presence of at least two of the listed symptoms. ‘Recovered’ was defined by self-reporting. Patients were placed in multiple groups if they experienced a combination of symptoms.
Matched nasal fluid and sputum samples were prospectively collected from a subgroup of convalescent patients approximately 6 months after hospitalization via the PHOSP-COVID study. Nasal and bronchoalveolar lavage fluid (BALF) collected from healthy volunteers before the COVID-19 pandemic were used as controls (Supplementary Table 4 ). Written consent was obtained for all individuals and ethical approvals were given by London–Harrow Research Ethics Committee (13/LO/1899) for the collection of nasal samples and the Health Research Authority London–Fulham Research Ethics Committee (IRAS project ID 154109; references 14/LO/1023, 10/H0711/94 and 11/LO/1826) for BALF samples.
Ethylenediaminetetraacetic acid plasma was collected from whole blood taken by venepuncture and frozen at −80 °C as previously described 7 , 66 . Nasal fluid was collected using a NasosorptionTM FX·I device (Hunt Developments), which uses a synthetic absorptive matrix to collect concentrated nasal fluid. Samples were eluted and stored as previously described 67 . Sputum samples were collected via passive expectoration and frozen at −80 °C without the addition of buffers. Sputum samples from convalescent individuals were compared with BALF from healthy SARS-CoV-2-naive controls, collected before the pandemic. BALF samples were used to act as a comparison for lower respiratory tract samples since passively expectorated sputum from healthy SARS-CoV-2-naive individuals was not available. BALF samples were obtained by instillation and recovery of up to 240 ml of normal saline via a fiberoptic bronchoscope. BALF was filtered through 100 µM strainers into sterile 50 ml Falcon tubes, then centrifuged for 10 min at 400 g at 4 °C. The resulting supernatant was transferred into sterile 50 ml Falcon tubes and frozen at −80 °C until use. The full methods for BALF collection and processing have been described previously 68 , 69 .
Immunoassays
To determine inflammatory signatures that associated with symptom outcomes, plasma samples were analyzed on an Olink Explore 384 Inflammation panel 70 . Supplementary Table 8 (Appendix 1 ) lists all the analytes measured. To ensure the validity of results, samples were run in a single batch with the use of negative controls, plate controls in triplicate and repeated measurement of patient samples between plates in duplicate. Samples were randomized between plates according to site and sample collection date. Randomization between plates was blind to LC/recovered outcome. Data were first normalized to an internal extension control that was included in each sample well. Plates were standardized by normalizing to interplate controls, run in triplicate on each plate. Each plate contained a minimum of four patient samples, which were duplicates on another plate; these duplicate pairs allowed any plate to be linked to any other through the duplicates. Data were then intensity normalized across all cohort samples. Finally, Olink results underwent quality control processing and samples or analytes that did not reach quality control standards were excluded. Final normalized relative protein quantities were reported as log 2 NPX values.
To further validate our findings, we performed conventional electrochemiluminescence (ECL) assays and enzyme-linked immunosorbent assay for Olink mediators that were associated with symptom outcome ( Supplementary Methods ). Contemporaneously collected plasma samples were available from 58 individuals. Like most omics platforms, Olink measures relative quantities, so perfect agreement with conventional assays that measure absolute concentrations is not expected.
Sputum samples were thawed before analysis and sputum plugs were extracted with the addition of 0.1% dithiothreitol creating a one in two sample dilution, as previously described 71 . SARS-CoV-2 S and N proteins were measured by ECL S-plex assay at a fixed dilution of one in two (Mesoscale Diagnostics), as per the manufacturers protocol 72 . Control BALF samples were thawed and measured on the same plate, neat. The S-plex assay is highly sensitive in detecting viral antigen in respiratory tract samples 73 .
Nasal cytokines were measured by ECL (mesoscale discovery) and Luminex bead multiplex assays (Biotechne). The full methods and list of analytes are detailed in Supplementary Methods .
Statistics and reproducibility
Clinical data was collected via the PHOSP REDCap database, to which access is available under reasonable request as per the data sharing statement in the manuscript. All analyses were performed within the Outbreak Data Analysis Platform (ODAP). All data and code can be accessed using information in the ‘Data sharing’ and ‘Code sharing’ statements at the end of the manuscript. No statistical method was used to predetermine sample size. Data distribution was assumed to be normal but this was not formally tested. Olink assays and immunoassays were randomized and investigators were blinded to outcomes.
To determine protein signatures that associated with each symptom outcome, a ridge PLR was used. PLR shrinks coefficients to account for combined effects within high-dimensional data, preventing false discovery while managing multicollinearity 34 . Thus, PLR was chosen a priori as the most appropriate model to assess associations between a large number of explanatory variables (that may work together to mediate effects) and symptom outcome 34 , 62 , 70 , 74 . In keeping with our aim to perform an unbiased exploration of inflammatory process, the model alpha was set to zero, facilitating regularization without complete penalization of any mediator. This enabled review of all possible mediators that might associate with LC 62 .
A 50 repeats tenfold nested cross-validation was used to select the optimal lambda for each model and assess its accuracy (Extended Data Fig. 1 ). The performance of the cognitive impairment model was influenced by the imbalance in size of the symptom group ( n = 60) relative to recovered ( n = 250). The model was weighted to account for this imbalance resulting in a sensitivity of 0.98, indicating its validity. We have expanded on the model performance and validation approaches in Supplementary Information .
Age, sex, acute disease severity and preexisting comorbidities were included as covariates in the PLR analysis (Supplementary Tables 1 and 3 ). Covariates were selected a priori using features reported to influence the risk of LC and inflammatory responses 1 , 39 , 64 , 75 . Ethnicity was not included since it has been shown not to predict symptom outcome in this cohort 64 . Individuals with missing data were excluded from the regression analysis. Each symptom group was compared with the ‘recovered’ group. The model coefficients of each covariate were converted into ORs for each outcome and visualized in a forest plot, after removing variables associated with regularized OR between 0.98 and 1.02 or in cases where most variables fell outside of this range, using mediators associated with the highest decile of coefficients either side of this range. This enabled exclusion of mediators with effect sizes that were unlikely to have clinical or mechanistic importance since the ridge PLR shrinks and orders coefficients according to their relative importance rather than making estimates with standard error. Thus, confidence intervals cannot be appropriately derived from PLR, and forest plot error bars were calculated using the median accuracy of the model generated by the nested cross-validation. To verify observations made through PLR analysis, we also performed an unadjusted PLR, an unadjusted logistic regression and a PLS analysis. Univariate analyses using Wilcoxon signed-rank test was also performed (Supplementary Table 8 , Appendix 1 ). Analyses were performed in R version 4.2.0 using ‘data.table v1.14.2’, ‘EnvStats v2.7.0’ ‘tidyverse v1.3.2’, ‘lme4 v1.1-32’, ‘caret v6.0-93’, ‘glmnet v4.1-6’, ‘mdatools v0.14.0’, ‘ggpubbr v0.4.0’ and ‘ggplot2 v3.3.6’ packages.
To further investigate the relationship between proteins elevated in each symptom group, we performed a correlation network analysis using Spearman’s rank correlation coefficient and false discovery rate (FDR) thresholding. The mediators visualized in the PLR forest plots, which were associated with cardiorespiratory symptoms, fatigue, anxiety/depression GI symptoms and cognitive impairment were used, respectively. Analyses were performed in R version 4.2.0 using ‘bootnet v1.5.6 ’ and ‘qgraph v1.9.8 ’ packages.
To determine whether differences in protein levels between men and women related to hormonal differences, we divided each symptom group into premenopausal and postmenopausal groups using an age cutoff of 50 years old. Differences between sexes in each group were determined using the Wilcoxon signed-rank test. To understand whether antigen persistence contributed to inflammation in adults with LC, the median viral antigen concentration from sputum/BALF samples and cytokine concentrations from nasal samples were compared using the Wilcoxon signed-rank test. All tests were two-tailed and statistical significance was defined as a P value < 0.05 after adjustment for FDR ( q -value of 0.05). Analyses were performed in R version 4.2.0 using ‘bootnet v1.5.6’ and ‘qgraph v1.9.8’ packages.
Extended Data Fig. 10 was made using Biorender, accessed at www.biorender.com .
Reporting summary
Further information on research design is available in the Nature Portfolio Reporting Summary linked to this article.
Data availability
This is an open access article under the CC BY 4.0 license.
The PHOSP-COVID protocol, consent form, definition and derivation of clinical characteristics and outcomes, training materials, regulatory documents, information about requests for data access, and other relevant study materials are available online at ref. 76 . Access to these materials can be granted by contacting [email protected] and [email protected].
The ISARIC4C protocol, data sharing and publication policy are available at https://isaric4c.net . ISARIC4C’s Independent Data and Material Access Committee welcomes applications for access to data and materials ( https://isaric4c.net ).
The datasets used in the study contain extensive clinical information at an individual level that prevent them from being deposited in an public depository due to data protection policies of the study. Study data can only be accessed via the ODAP, a protected research environment. All data used in this study are available within ODAP and accessible under reasonable request. Data access criteria and information about how to request access is available online at ref. 76 . If criteria are met and a request is made, access can be gained by signing the eDRIS user agreement.
Code availability
Code was written within the ODAP, using R v4.2.0 and publicly available packages (‘data.table v1.14.2’, ‘EnvStats v2.7.0’, ‘tidyverse v1.3.2’, ‘lme4 v1.1-32’, ‘caret v6.0-93’, ‘glmnet v4.1-6’, ‘mdatools v0.14.0’, ‘ggpubbr v0.4.0’, ‘ggplot2 v3.3.6’, ‘bootnet v1.5.6’ and ‘qgraph v1.9.8’ packages). No new algorithms or functions were created and code used in-built functions in listed packages available on CRAN. The code used to generate data and to analyze data is publicly available at https://github.com/isaric4c/wiki/wiki/ISARIC ; https://github.com/SurgicalInformatics/cocin_cc and https://github.com/ClaudiaEfstath/PHOSP_Olink_NatImm .
Davis, H. E., McCorkell, L., Vogel, J. M. & Topol, E. J. Long COVID: major findings, mechanisms and recommendations. Nat. Rev. Microbiol. 21 , 133–146 (2023).
Article CAS PubMed PubMed Central Google Scholar
Antonelli, M., Pujol, J. C., Spector, T. D., Ourselin, S. & Steves, C. J. Risk of long COVID associated with delta versus omicron variants of SARS-CoV-2. Lancet 399 , 2263–2264 (2022).
Talla, A. et al. Persistent serum protein signatures define an inflammatory subcategory of long COVID. Nat. Commun. 14 , 3417 (2023).
Captur, G. et al. Plasma proteomic signature predicts who will get persistent symptoms following SARS-CoV-2 infection. EBioMedicine 85 , 104293 (2022).
Scott, N. A. et al. Monocyte migration profiles define disease severity in acute COVID-19 and unique features of long COVID. Eur. Respir. J. https://doi.org/10.1183/13993003.02226-2022 (2023).
Klein, J. et al. Distinguishing features of Long COVID identified through immune profiling. Nature https://doi.org/10.1038/s41586-023-06651-y (2023).
Article PubMed PubMed Central Google Scholar
Evans, R. A. et al. Clinical characteristics with inflammation profiling of long COVID and association with 1-year recovery following hospitalisation in the UK: a prospective observational study. Lancet Respir. Med . 10 , 761–775 (2022).
Article CAS Google Scholar
Houchen-Wolloff, L. et al. Joint patient and clinician priority setting to identify 10 key research questions regarding the long-term sequelae of COVID-19. Thorax 77 , 717–720 (2022).
Article PubMed Google Scholar
Marshall, J. C. et al. A minimal common outcome measure set for COVID-19 clinical research. Lancet Infect. Dis. 20 , e192–e197 (2020).
Post COVID-19 condition (long COVID). World Health Organization https://www.who.int/europe/news-room/fact-sheets/item/post-covid-19-condition#:~:text=Definition,months%20with%20no%20other%20explanation (2022).
Peters, V. A., Joesting, J. J. & Freund, G. G. IL-1 receptor 2 (IL-1R2) and its role in immune regulation. Brain Behav. Immun. 32 , 1–8 (2013).
Article CAS PubMed Google Scholar
Luo, Z. et al. Monocytes augment inflammatory responses in human aortic valve interstitial cells via β2-integrin/ICAM-1-mediated signaling. Inflamm. Res. 71 , 681–694 (2022).
Bendall, L. J. & Bradstock, K. F. G-CSF: from granulopoietic stimulant to bone marrow stem cell mobilizing agent. Cytokine Growth Factor Rev. 25 , 355–367 (2014).
Ma, Y. J. et al. Soluble collectin-12 (CL-12) is a pattern recognition molecule initiating complement activation via the alternative pathway. J. Immunol. 195 , 3365–3373 (2015).
Laursen, N. S. et al. Functional and structural characterization of a potent C1q inhibitor targeting the classical pathway of the complement system. Front. Immunol. 11 , 1504 (2020).
Dejanovic, B. et al. Complement C1q-dependent excitatory and inhibitory synapse elimination by astrocytes and microglia in Alzheimer’s disease mouse models. Nat. Aging 2 , 837–850 (2022).
Xue, G., Hua, L., Zhou, N. & Li, J. Characteristics of immune cell infiltration and associated diagnostic biomarkers in ulcerative colitis: results from bioinformatics analysis. Bioengineered 12 , 252–265 (2021).
He, T. et al. Integrative computational approach identifies immune‐relevant biomarkers in ulcerative colitis. FEBS Open Bio. 12 , 500–515 (2022).
Sundin, J. et al. Fecal chromogranins and secretogranins are linked to the fecal and mucosal intestinal bacterial composition of IBS patients and healthy subjects. Sci. Rep. 8 , 16821 (2018).
Kriebel, M., Wuchter, J., Trinks, S. & Volkmer, H. Neurofascin: a switch between neuronal plasticity and stability. Int. J. Biochem. Cell Biol. 44 , 694–697 (2012).
Woo, W.-M. et al. The C. elegans F-spondin family protein SPON-1 maintains cell adhesion in neural and non-neural tissues. Development 135 , 2747–2756 (2008).
Yadati, T., Houben, T., Bitorina, A. & Shiri-Sverdlov, R. The ins and outs of cathepsins: physiological function and role in disease management. Cells 9 , 1679 (2020).
Taquet, M. et al. Acute blood biomarker profiles predict cognitive deficits 6 and 12 months after COVID-19 hospitalization. Nat. Med. https://doi.org/10.1038/s41591-023-02525-y (2023).
Siggins, M. K. et al. Alternative pathway dysregulation in tissues drives sustained complement activation and predicts outcome across the disease course in COVID‐19. Immunology 168 , 473–492 (2023).
Pol, J. G., Caudana, P., Paillet, J., Piaggio, E. & Kroemer, G. Effects of interleukin-2 in immunostimulation and immunosuppression. J. Exp. Med. 217 , e20191247 (2020).
Zhang, Y., Liu, Q., Yang, S. & Liao, Q. CD58 immunobiology at a glance. Front. Immunol. 12 , 705260 (2021).
Demydchuk, M. et al. Insights into Hunter syndrome from the structure of iduronate-2-sulfatase. Nat. Commun. 8 , 15786 (2017).
Wang, Z. et al. DNER promotes epithelial–mesenchymal transition and prevents chemosensitivity through the Wnt/β-catenin pathway in breast cancer. Cell Death Dis. 11 , 642 (2020).
Bonilla, H. et al. Therapeutic trials for long COVID-19: a call to action from the interventions taskforce of the RECOVER initiative. Front. Immunol. 14 , 1129459 (2023).
Wik, L. et al. Proximity extension assay in combination with next-generation sequencing for high-throughput proteome-wide analysis. Mol. Cell. Proteomics 20 , 100168 (2021).
Measuring protein biomarkers with Olink—technical comparisons and orthogonal validation. Olink Proteomics https://www.olink.com/content/uploads/2021/09/olink-technical-comparisons-and-orthogonal-validation-1118-v2.0.pdf (2021).
COVID-19 rapid guideline: managing the long-term effects of COVID-19. National Institute for Health and Care Excellence (NICE), Scottish Intercollegiate Guidelines Network (SIGN) and Royal College of General Practitioners (RCGP) https://www.nice.org.uk/guidance/ng188/resources/covid19-rapid-guideline-managing-the-longterm-effects-of-covid19-pdf-51035515742 (2022).
Long COVID or post-COVID conditions. Centers for Disease Control and Prevention https://www.cdc.gov/coronavirus/2019-ncov/long-term-effects/index.html#:~:text=Long%20COVID%20is%20broadly%20defined,after%20acute%20COVID%2D19%20infection (2023).
Firinguetti, L., Kibria, G. & Araya, R. Study of partial least squares and ridge regression methods. Commun. Stat. Simul. Comput 46 , 6631–6644 (2017).
Article Google Scholar
Mortensen, L. J., Kreiner-Moller, E., Hakonarson, H., Bonnelykke, K. & Bisgaard, H. The PCDH1 gene and asthma in early childhood. Eur. Respir. J. 43 , 792–800 (2014).
Tong, Y. et al. The RNFT2/IL-3Rα axis regulates IL-3 signaling and innate immunity. JCI Insight 5 , e133652 (2020).
Wu, Y. et al. Effect of ISM1 on the immune microenvironment and epithelial-mesenchymal transition in colorectal cancer. Front. Cell Dev. Biol. 9 , 681240 (2021).
Luo, G. G. & Ou, J. J. Oncogenic viruses and cancer. Virol. Sin. 30 , 83–84 (2015).
Klein, S. L. & Flanagan, K. L. Sex differences in immune responses. Nat. Rev. Immunol. 16 , 626–638 (2016).
Gaebler, C. et al. Evolution of antibody immunity to SARS-CoV-2. Nature 591 , 639–644 (2021).
Bussani, R. et al. Persistent SARS‐CoV‐2 infection in patients seemingly recovered from COVID‐19. J. Pathol. 259 , 254–263 (2023).
Woodruff, M. C. et al. Chronic inflammation, neutrophil activity, and autoreactivity splits long COVID. Nat. Commun. 14 , 4201 (2023).
Lammi, V. et al. Genome-wide association study of long COVID. Preprint at medRxiv https://doi.org/10.1101/2023.06.29.23292056 (2023).
Ismailova, A. et al. Identification of a forkhead box protein transcriptional network induced in human neutrophils in response to inflammatory stimuli. Front. Immunol. 14 , 1123344 (2023).
Beurskens, F. J., van Schaarenburg, R. A. & Trouw, L. A. C1q, antibodies and anti-C1q autoantibodies. Mol. Immunol. 68 , 6–13 (2015).
Cervia-Hasler, C. et al. Persistent complement dysregulation with signs of thromboinflammation in active long Covid. Science 383 , eadg7942 (2024).
Morgan, B. P. & Harris, C. L. Complement, a target for therapy in inflammatory and degenerative diseases. Nat. Rev. Drug Discov. 14 , 857–877 (2015).
Toshner, M. R. et al. Apixaban following discharge in hospitalised adults with COVID-19: preliminary results from a multicentre, open-label, randomised controlled platform clinical trial. Preprint at medRxiv , https://doi.org/10.1101/2022.12.07.22283175 (2022).
Su, Y. et al. Multiple early factors anticipate post-acute COVID-19 sequelae. Cell 185 , 881–895.e20 (2022).
Branchfield, K. et al. Pulmonary neuroendocrine cells function as airway sensors to control lung immune response. Science 351 , 707–710 (2016).
Rivera-Torruco, G. et al. Isthmin 1 identifies a subset of lung hematopoietic stem cells and it is associated with systemic inflammation. J. Immunol. 202 , 118.18 (2019).
Swank, Z. et al. Persistent circulating severe acute respiratory syndrome coronavirus 2 spike is associated with post-acute coronavirus disease 2019 sequelae. Clin. Infect. Dis. 76 , e487–e490 (2023).
Peluso, M. J. et al. Chronic viral coinfections differentially affect the likelihood of developing long COVID. J. Clin. Invest. 133 , e163669 (2023).
Bellocchi, C. et al. The interplay between autonomic nervous system and inflammation across systemic autoimmune diseases. Int. J. Mol. Sci. 23 , 2449 (2022).
Raman, B. et al. Multiorgan MRI findings after hospitalisation with COVID-19 in the UK (C-MORE): a prospective, multicentre, observational cohort study. Lancet Respir. Med 11 , 1003–1019 (2023).
Fernández-Castañeda, A. et al. Mild respiratory COVID can cause multi-lineage neural cell and myelin dysregulation. Cell 185 , 2452–2468.e16 (2022).
Dantzer, R., O’Connor, J. C., Freund, G. G., Johnson, R. W. & Kelley, K. W. From inflammation to sickness and depression: when the immune system subjugates the brain. Nat. Rev. Neurosci. 9 , 46–56 (2008).
Broughton, S. E. et al. Dual mechanism of interleukin-3 receptor blockade by an anti-cancer antibody. Cell Rep. 8 , 410–419 (2014).
Ley, K., Miller, Y. I. & Hedrick, C. C. Monocyte and macrophage dynamics during atherogenesis. Arterioscler. Thromb. Vasc. Biol. 31 , 1506–1516 (2011).
Iosef, C. et al. Plasma proteome of long-COVID patients indicates HIF-mediated vasculo-proliferative disease with impact on brain and heart function. J. Transl. Med. 21 , 377 (2023).
Santopaolo, M. et al. Prolonged T-cell activation and long COVID symptoms independently associate with severe COVID-19 at 3 months. eLife 12 , e85009 (2023).
Friedman, J., Hastie, T. & Tibshirani, R. Regularization paths for generalized linear models via coordinate descent. J. Stat. Softw. 33 , 1–22 (2010).
Voiriot, G. et al. Chronic critical illness and post-intensive care syndrome: from pathophysiology to clinical challenges. Ann. Intensive Care 12 , 58 (2022).
Evans, R. A. et al. Physical, cognitive, and mental health impacts of COVID-19 after hospitalisation (PHOSP-COVID): a UK multicentre, prospective cohort study. Lancet Respir. Med 9 , 1275–1287 (2021).
Docherty, A. B. et al. Features of 20,133 UK patients in hospital with covid-19 using the ISARIC WHO clinical characterisation protocol: prospective observational cohort study. BMJ https://doi.org/10.1136/bmj.m1985 (2020).
Elneima, O. et al. Cohort profile: post-hospitalisation COVID-19 study (PHOSP-COVID). Preprint at medRxiv https://doi.org/10.1101/2023.05.08.23289442 (2023).
Liew, F. et al. SARS-CoV-2-specific nasal IgA wanes 9 months after hospitalisation with COVID-19 and is not induced by subsequent vaccination. EBioMedicine 87 , 104402 (2023).
Ascough, S. et al. Divergent age-related humoral correlates of protection against respiratory syncytial virus infection in older and young adults: a pilot, controlled, human infection challenge model. Lancet Healthy Longev. 3 , e405–e416 (2022).
Guvenel, A. et al. Epitope-specific airway-resident CD4+ T cell dynamics during experimental human RSV infection. J. Clin. Invest. 130 , 523–538 (2019).
Article PubMed Central Google Scholar
Greenwood, C. J. et al. A comparison of penalised regression methods for informing the selection of predictive markers. PLoS ONE 15 , e0242730 (2020).
Higham, A. et al. Leukotriene B4 levels in sputum from asthma patients. ERJ Open Res. 2 , 00088–02015 (2016).
SARS-CoV-2 spike kit. MSD https://www.mesoscale.com/~/media/files/product%20inserts/s-plex%20sars-cov-2%20spike%20kit%20product%20insert.pdf (2023).
Ren, A. et al. Ultrasensitive assay for saliva-based SARS-CoV-2 antigen detection. Clin. Chem. Lab. Med. 60 , 771–777 (2022).
Breheny, P. & Huang, J. Penalized methods for bi-level variable selection. Stat. Interface 2 , 369–380 (2009).
Thwaites, R. S. et al. Inflammatory profiles across the spectrum of disease reveal a distinct role for GM-CSF in severe COVID-19. Sci. Immunol. 6 , eabg9873 (2021).
Resources. PHOSP-COVID https://phosp.org/resource/ (2022).
Download references
Acknowledgements
This research used data assets made available by ODAP as part of the Data and Connectivity National Core Study, led by Health Data Research UK in partnership with the Office for National Statistics and funded by UK Research and Innovation (grant ref. MC_PC_20058). This work is supported by the following grants: the PHOSP-COVD study is jointly funded by UK Research and Innovation and National Institute of Health and Care Research (NIHR; grant references MR/V027859/1 and COV0319). ISARIC4C is supported by grants from the National Institute for Health and Care Research (award CO-CIN-01) and the MRC (grant MC_PC_19059) Liverpool Experimental Cancer Medicine Centre provided infrastructure support for this research (grant reference C18616/A25153). Other grants that have supported this work include the UK Coronavirus Immunology Consortium (funder reference 1257927), the Imperial Biomedical Research Centre (NIHR Imperial BRC, grant IS-BRC-1215-20013), the Health Protection Research Unit in Respiratory Infections at Imperial College London and NIHR Health Protection Research Unit in Emerging and Zoonotic Infections at University of Liverpool, both in partnership with Public Health England, (NIHR award 200907), Wellcome Trust and Department for International Development (215091/Z/18/Z), Health Data Research UK (grant code 2021.0155), MRC (grant code MC_UU_12014/12) and NIHR Clinical Research Network for providing infrastructure support for this research. We also acknowledge the support of the MRC EMINENT Network (MR/R502121/1), which is cofunded by GSK, the Comprehensive Local Research Networks, the MRC HIC-Vac network (MR/R005982/1) and the RSV Consortium in Europe Horizon 2020 Framework Grant 116019. F.L. is supported by an MRC clinical training fellowship (award MR/W000970/1). C.E. is funded by NIHR (grant P91258-4). L.-P.H. is supported by Oxford NIHR Biomedical Research Centre. A.A.R.T. is supported by a British Heart Foundation (BHF) Intermediate Clinical Fellowship (FS/18/13/33281). S.L.R.-J. receives support from UK Research and Innovation (UKRI), Global Challenges Research Fund (GCRF), Rosetrees Trust, British HIV association (BHIVA), European & Developing Countries Clinical Trials Partnership (EDCTP) and Globvac. J.D.C. has grants from AstraZeneca, Boehringer Ingelheim, GSK, Gilead Sciences, Grifols, Novartis and Insmed. R.A.E. holds a NIHR Clinician Scientist Fellowship (CS-2016-16-020). A. Horsley is currently supported by UK Research and Innovation, NIHR and NIHR Manchester BRC. B.R. receives support from BHF Oxford Centre of Research Excellence, NIHR Oxford BRC and MRC. D.G.W. is supported by an NIHR Advanced Fellowship. A. Ho has received support from MRC and for the Coronavirus Immunology Consortium (MR/V028448/1). L.T. is supported by the US Food and Drug Administration Medical Countermeasures Initiative contract 75F40120C00085 and the National Institute for Health Research Health Protection Research Unit in Emerging and Zoonotic Infections (NIHR200907) at the University of Liverpool in partnership with UK Health Security Agency (UK-HSA), in collaboration with Liverpool School of Tropical Medicine and the University of Oxford. L.V.W. has received support from UKRI, GSK/Asthma and Lung UK and NIHR for this study. M.G.S. has received support from NIHR UK, MRC UK and Health Protection Research Unit in Emerging and Zoonotic Infections, University of Liverpool. J.K.B. is supported by the Wellcome Trust (223164/Z/21/Z) and UKRI (MC_PC_20004, MC_PC_19025, MC_PC_1905, MRNO2995X/1 and MC_PC_20029). The funders were not involved in the study design, interpretation of data or writing of this manuscript. The views expressed are those of the authors and not necessarily those of the Department of Health and Social Care (DHSC), the Department for International Development (DID), NIHR, MRC, the Wellcome Trust, UK-HSA, the National Health Service or the Department of Health. P.J.M.O. is supported by a NIHR Senior Investigator Award (award 201385). We thank all the participants and their families. We thank the many research administrators, health-care and social-care professionals who contributed to setting up and delivering the PHOSP-COVID study at all of the 65 NHS trusts/health boards and 25 research institutions across the United Kingdom, as well as those who contributed to setting up and delivering the ISARIC4C study at 305 NHS trusts/health boards. We also thank all the supporting staff at the NIHR Clinical Research Network, Health Research Authority, Research Ethics Committee, Department of Health and Social Care, Public Health Scotland and Public Health England. We thank K. Holmes at the NIHR Office for Clinical Research Infrastructure for her support in coordinating the charities group. The PHOSP-COVID industry framework was formed to provide advice and support in commercial discussions, and we thank the Association of the British Pharmaceutical Industry as well the NIHR Office for Clinical Research Infrastructure for coordinating this. We are very grateful to all the charities that have provided insight to the study: Action Pulmonary Fibrosis, Alzheimer’s Research UK, Asthma and Lung UK, British Heart Foundation, Diabetes UK, Cystic Fibrosis Trust, Kidney Research UK, MQ Mental Health, Muscular Dystrophy UK, Stroke Association Blood Cancer UK, McPin Foundations and Versus Arthritis. We thank the NIHR Leicester Biomedical Research Centre patient and public involvement group and Long Covid Support. We also thank G. Khandaker and D. C. Newcomb who provided valuable feedback on this work. Extended Data Fig. 10 was created using Biorender.
Author information
These authors contributed equally: Felicity Liew, Claudia Efstathiou, Ryan S. Thwaites, Peter J. M. Openshaw.
Authors and Affiliations
National Heart and Lung Institute, Imperial College London, London, UK
Felicity Liew, Claudia Efstathiou, Sara Fontanella, Dawid Swieboda, Jasmin K. Sidhu, Stephanie Ascough, Onn Min Kon, Luke S. Howard, Jennifer K. Quint, Christopher Chiu, Ryan S. Thwaites, Peter J. M. Openshaw, Jake Dunning & Peter J. M. Openshaw
Institute for Lung Health, Leicester NIHR Biomedical Research Centre, University of Leicester, Leicester, UK
Matthew Richardson, Ruth Saunders, Olivia C. Leavy, Omer Elneima, Hamish J. C. McAuley, Amisha Singapuri, Marco Sereno, Victoria C. Harris, Neil J. Greening, Rachael A. Evans, Louise V. Wain, Christopher Brightling & Ananga Singapuri
NIHR Health Protection Research Unit in Emerging and Zoonotic Infections, Institute of Infection, Veterinary and Ecological Sciences, University of Liverpool, Liverpool, UK
Shona C. Moore, Daniel G. Wootton, Malcolm G. Semple, Lance Turtle, William A. Paxton & Georgios Pollakis
The Imperial Clinical Respiratory Research Unit, Imperial College NHS Trust, London, UK
Noura Mohamed
Cardiovascular Research Team, Imperial College Healthcare NHS Trust, London, UK
Jose Nunag & Clara King
Department of Population Health Sciences, University of Leicester, Leicester, UK
Olivia C. Leavy, Louise V. Wain & Beatriz Guillen-Guio
NIHR Leicester Biomedical Research Centre, University of Leicester, Leicester, UK
Aarti Shikotra
Centre for Exercise and Rehabilitation Science, NIHR Leicester Biomedical Research Centre-Respiratory, University of Leicester, Leicester, UK
Linzy Houchen-Wolloff
Usher Institute, University of Edinburgh, Edinburgh, UK
Nazir I. Lone, Luke Daines, Annemarie B. Docherty, Nazir I. Lone, Matthew Thorpe, Annemarie B. Docherty, Thomas M. Drake, Cameron J. Fairfield, Ewen M. Harrison, Stephen R. Knight, Kenneth A. Mclean, Derek Murphy, Lisa Norman, Riinu Pius & Catherine A. Shaw
Centre for Medical Informatics, The Usher Institute, University of Edinburgh, Edinburgh, UK
Matthew Thorpe, Annemarie B. Docherty, Ewen M. Harrison, J. Kenneth Baillie, Sarah L. Rowland-Jones, A. A. Roger Thompson & Thushan de Silva
Department of Infection, Immunity and Cardiovascular Disease, University of Sheffield, Sheffield, UK
A. A. Roger Thompson, Sarah L. Rowland-Jones, Thushan I. de Silva & James D. Chalmers
University of Dundee, Ninewells Hospital and Medical School, Dundee, UK
James D. Chalmers & Ling-Pei Ho
MRC Human Immunology Unit, University of Oxford, Oxford, UK
Ling-Pei Ho & Alexander Horsley
Division of Infection, Immunity and Respiratory Medicine, Faculty of Biology, Medicine and Health, University of Manchester, Manchester, UK
Alexander Horsley & Betty Raman
Radcliffe Department of Medicine, University of Oxford, Oxford, UK
Betty Raman & Krisnah Poinasamy
Asthma + Lung UK, London, UK
Krisnah Poinasamy & Michael Marks
Department of Clinical Research, London School of Hygiene and Tropical Medicine, London, UK
Michael Marks
Hospital for Tropical Diseases, University College London Hospital, London, UK
Division of Infection and Immunity, University College London, London, UK
Michael Marks & Mahdad Noursadeghi
MRC Centre for Virus Research, School of Infection and Immunity, University of Glasgow, Glasgow, UK
Antonia Ho & William Greenhalf
Institute of Systems, Molecular and Integrative Biology, University of Liverpool, Liverpool, UK
William Greenhalf & J. Kenneth Baillie
The Roslin Institute, University of Edinburgh, Edinburgh, UK
J. Kenneth Baillie, J. Kenneth Baillie, Sara Clohisey, Fiona Griffiths, Ross Hendry, Andrew Law & Wilna Oosthuyzen
Pandemic Science Hub, University of Edinburgh, Edinburgh, UK
J. Kenneth Baillie
The Pandemic Institute, University of Liverpool, Liverpool, UK
Malcolm G. Semple & Lance Turtle
University of Manchester, Manchester, UK
Kathryn Abel, Perdita Barran, H. Chinoy, Bill Deakin, M. Harvie, C. A. Miller, Stefan Stanel & Drupad Trivedi
Intensive Care Unit, Royal Infirmary of Edinburgh, Edinburgh, UK
Kathryn Abel & J. Kenneth Baillie
North Bristol NHS Trust and University of Bristol, Bristol, UK
H. Adamali, David Arnold, Shaney Barratt, A. Dipper, Sarah Dunn, Nick Maskell, Anna Morley, Leigh Morrison, Louise Stadon, Samuel Waterson & H. Welch
University of Edinburgh, Manchester, UK
Davies Adeloye, D. E. Newby, Riinu Pius, Igor Rudan, Manu Shankar-Hari, Catherine Sudlow, Sarah Walmsley & Bang Zheng
King’s College Hospital NHS Foundation Trust and King’s College London, London, UK
Oluwaseun Adeyemi, Rita Adrego, Hosanna Assefa-Kebede, Jonathon Breeze, S. Byrne, Pearl Dulawan, Amy Hoare, Caroline Jolley, Abigail Knighton, M. Malim, Sheetal Patale, Ida Peralta, Natassia Powell, Albert Ramos, K. Shevket, Fabio Speranza & Amelie Te
Guy’s and St Thomas’ NHS Foundation Trust, London, UK
Laura Aguilar Jimenez, Gill Arbane, Sarah Betts, Karen Bisnauthsing, A. Dewar, Nicholas Hart, G. Kaltsakas, Helen Kerslake, Murphy Magtoto, Philip Marino, L. M. Martinez, Marlies Ostermann, Jennifer Rossdale & Teresa Solano
Royal Free London NHS Foundation Trust, London, UK
Shanaz Ahmad, Simon Brill, John Hurst, Hannah Jarvis, C. Laing, Lai Lim, S. Mandal, Darwin Matila, Olaoluwa Olaosebikan & Claire Singh
University Hospital Birmingham NHS Foundation Trust and University of Birmingham, Birmingham, UK
N. Ahmad Haider, Catherine Atkin, Rhiannon Baggott, Michelle Bates, A. Botkai, Anna Casey, B. Cooper, Joanne Dasgin, Camilla Dawson, Katharine Draxlbauer, N. Gautam, J. Hazeldine, T. Hiwot, Sophie Holden, Karen Isaacs, T. Jackson, Vicky Kamwa, D. Lewis, Janet Lord, S. Madathil, C. McGee, K. Mcgee, Aoife Neal, Alex Newton-Cox, Joseph Nyaboko, Dhruv Parekh, Z. Peterkin, H. Qureshi, Liz Ratcliffe, Elizabeth Sapey, J. Short, Tracy Soulsby, J. Stockley, Zehra Suleiman, Tamika Thompson, Maximina Ventura, Sinead Walder, Carly Welch, Daisy Wilson, S. Yasmin & Kay Por Yip
Stroke Association, London, UK
Rubina Ahmed & Richard Francis
University College London Hospital and University College London, London, UK
Nyarko Ahwireng, Dongchun Bang, Donna Basire, Jeremy Brown, Rachel Chambers, A. Checkley, R. Evans, M. Heightman, T. Hillman, Joseph Jacob, Roman Jastrub, M. Lipman, S. Logan, D. Lomas, Marta Merida Morillas, Hannah Plant, Joanna Porter, K. Roy & E. Wall
Oxford University Hospitals NHS Foundation Trust and University of Oxford, Oxford, UK
Mark Ainsworth, Asma Alamoudi, Angela Bloss, Penny Carter, M. Cassar, Jin Chen, Florence Conneh, T. Dong, Ranuromanana Evans, V. Ferreira, Emily Fraser, John Geddes, F. Gleeson, Paul Harrison, May Havinden-Williams, P. Jezzard, Ivan Koychev, Prathiba Kurupati, H. McShane, Clare Megson, Stefan Neubauer, Debby Nicoll, C. Nikolaidou, G. Ogg, Edmund Pacpaco, M. Pavlides, Yanchun Peng, Nayia Petousi, John Pimm, Najib Rahman, M. J. Rowland, Kathryn Saunders, Michael Sharpe, Nick Talbot, E. M. Tunnicliffe & C. Xie
St George’s University Hospitals NHS Foundation Trust, London, UK
Mariam Ali, Raminder Aul, A. Dunleavy, D. Forton, Mark Mencias, N. Msimanga, T. Samakomva, Sulman Siddique, Vera Tavoukjian & J. Teixeira
University Hospitals of Leicester NHS Trust and University of Leicester, Leicester, UK
M. Aljaroof, Natalie Armstrong, H. Arnold, Hnin Aung, Majda Bakali, M. Bakau, E. Baldry, Molly Baldwin, Charlotte Bourne, Michelle Bourne, Nigel Brunskill, P. Cairns, Liesel Carr, Amanda Charalambou, C. Christie, Melanie Davies, Enya Daynes, Sarah Diver, Rachael Dowling, Sarah Edwards, C. Edwardson, H. Evans, J. Finch, Sarah Glover, Nicola Goodman, Bibek Gooptu, Kate Hadley, Pranab Haldar, Beverley Hargadon, W. Ibrahim, L. Ingram, Kamlesh Khunti, A. Lea, D. Lee, Gerry McCann, P. McCourt, Teresa Mcnally, George Mills, Will Monteiro, Manish Pareek, S. Parker, Anne Prickett, I. N. Qureshi, A. Rowland, Richard Russell, Salman Siddiqui, Sally Singh, J. Skeemer, M. Soares, E. Stringer, T. Thornton, Martin Tobin, T. J. C. Ward, F. Woodhead, Tom Yates & A. J. Yousuf
University of Exeter, Exeter, UK
Louise Allan, Clive Ballard & Andrew McGovern
University of Leicester, Leicester, UK
Richard Allen, Michelle Bingham, Terry Brugha, Selina Finney, Rob Free, Don Jones, Claire Lawson, Daniel Lozano-Rojas, Gardiner Lucy, Alistair Moss, Elizabeta Mukaetova-Ladinska, Petr Novotny, Kimon Ntotsis, Charlotte Overton, John Pearl, Tatiana Plekhanova, M. Richardson, Nilesh Samani, Jack Sargant, Ruth Saunders, M. Sharma, Mike Steiner, Chris Taylor, Sarah Terry, C. Tong, E. Turner, J. Wormleighton & Bang Zhao
Liverpool University Hospitals NHS Foundation Trust and University of Liverpool, Liverpool, UK
Lisa Allerton, Ann Marie Allt, M. Beadsworth, Anthony Berridge, Jo Brown, Shirley Cooper, Andy Cross, Sylviane Defres, S. L. Dobson, Joanne Earley, N. French, Kera Hainey, Hayley Hardwick, Jenny Hawkes, Victoria Highett, Sabina Kaprowska, Angela Key, Lara Lavelle-Langham, N. Lewis-Burke, Gladys Madzamba, Flora Malein, Sophie Marsh, Chloe Mears, Lucy Melling, Matthew Noonan, L. Poll, James Pratt, Emma Richardson, Anna Rowe, Victoria Shaw, K. A. Tripp, Lilian Wajero, S. A. Williams-Howard, Dan Wootton & J. Wyles
Sherwood Forest Hospitals NHS Foundation Trust, Nottingham, UK
Lynne Allsop, Kaytie Bennett, Phil Buckley, Margaret Flynn, Mandy Gill, Camelia Goodwin, M. Greatorex, Heidi Gregory, Cheryl Heeley, Leah Holloway, Megan Holmes, John Hutchinson, Jill Kirk, Wayne Lovegrove, Terri Ann Sewell, Sarah Shelton, D. Sissons, Katie Slack, Susan Smith, D. Sowter, Sarah Turner, V. Whitworth & Inez Wynter
Nottingham University Hospitals NHS Trust and University of Nottingham, London, UK
Paula Almeida, Akram Hosseini, Robert Needham & Karen Shaw
Manchester University NHS Foundation Trust and University of Manchester, London, UK
Bashar Al-Sheklly, Cristina Avram, John Blaikely, M. Buch, N. Choudhury, David Faluyi, T. Felton, T. Gorsuch, Neil Hanley, Tracy Hussell, Zunaira Kausar, Natasha Odell, Rebecca Osbourne, Karen Piper Hanley, K. Radhakrishnan & Sue Stockdale
Imperial College London, London, UK
Danny Altmann, Anew Frankel, Luke S. Howard, Desmond Johnston, Liz Lightstone, Anne Lingford-Hughes, William Man, Steve McAdoo, Jane Mitchell, Philip Molyneaux, Christos Nicolaou, D. P. O’Regan, L. Price, Jennifer K. Quint, David Smith, Jonathon Valabhji, Simon Walsh, Martin Wilkins & Michelle Willicombe
Hampshire Hospitals NHS Foundation Trust, Basingstoke, UK
Maria Alvarez Corral, Ava Maria Arias, Emily Bevan, Denise Griffin, Jane Martin, J. Owen, Sheila Payne, A. Prabhu, Annabel Reed, Will Storrar, Nick Williams & Caroline Wrey Brown
British Heart Foundation, Birmingham, UK
Shannon Amoils
NHS Greater Glasgow and Clyde Health Board and University of Glasgow, Glasgow, UK
David Anderson, Neil Basu, Hannah Bayes, Colin Berry, Ammani Brown, Andrew Dougherty, K. Fallon, L. Gilmour, D. Grieve, K. Mangion, I. B. McInnes, A. Morrow, Kathryn Scott & R. Sykes
University of Oxford, Oxford, UK
Charalambos Antoniades, A. Bates, M. Beggs, Kamaldeep Bhui, Katie Breeze, K. M. Channon, David Clark, X. Fu, Masud Husain, Lucy Kingham, Paul Klenerman, Hanan Lamlum, X. Li, E. Lukaschuk, Celeste McCracken, K. McGlynn, R. Menke, K. Motohashi, T. E. Nichols, Godwin Ogbole, S. Piechnik, I. Propescu, J. Propescu, A. A. Samat, Z. B. Sanders, Louise Sigfrid & M. Webster
Belfast Health and Social Care Trust and Queen’s University Belfast, Belfast, UK
Cherie Armour, Vanessa Brown, John Busby, Bronwen Connolly, Thelma Craig, Stephen Drain, Liam Heaney, Bernie King, Nick Magee, E. Major, Danny McAulay, Lorcan McGarvey, Jade McGinness, Tunde Peto & Roisin Stone
Airedale NHS Foundation Trust, Keighley, UK
Lisa Armstrong, Brigid Hairsine, Helen Henson, Claire Kurasz, Alison Shaw & Liz Shenton
Wrightington Wigan and Leigh NHS Trust, Wigan, UK
A. Ashish, Josh Cooper & Emma Robinson
Leeds Teaching Hospitals and University of Leeds, Leeds, UK
Andrew Ashworth, Paul Beirne, Jude Clarke, C. Coupland, Matthhew Dalton, Clair Favager, Jodie Glossop, John Greenwood, Lucy Hall, Tim Hardy, Amy Humphries, Jennifer Murira, Dan Peckham, S. Plein, Jade Rangeley, Gwen Saalmink, Ai Lyn Tan, Elaine Wade, Beverley Whittam, Nicola Window & Janet Woods
University of Liverpool, Liverpool, UK
M. Ashworth, D. Cuthbertson, G. Kemp, Anne McArdle, Benedict Michael, Will Reynolds, Lisa Spencer, Ben Vinson, Katie A. Ahmed, Jane A. Armstrong, Milton Ashworth, Innocent G. Asiimwe, Siddharth Bakshi, Samantha L. Barlow, Laura Booth, Benjamin Brennan, Katie Bullock, Nicola Carlucci, Emily Cass, Benjamin W. A. Catterall, Jordan J. Clark, Emily A. Clarke, Sarah Cole, Louise Cooper, Helen Cox, Christopher Davis, Oslem Dincarslan, Alejandra Doce Carracedo, Chris Dunn, Philip Dyer, Angela Elliott, Anthony Evans, Lorna Finch, Lewis W. S. Fisher, Lisa Flaherty, Terry Foster, Isabel Garcia-Dorival, Philip Gunning, Catherine Hartley, Karl Holden, Anthony Holmes, Rebecca L. Jensen, Christopher B. Jones, Trevor R. Jones, Shadia Khandaker, Katharine King, Robyn T. Kiy, Chrysa Koukorava, Annette Lake, Suzannah Lant, Diane Latawiec, Lara Lavelle-Langham, Daniella Lefteri, Lauren Lett, Lucia A. Livoti, Maria Mancini, Hannah Massey, Nicole Maziere, Sarah McDonald, Laurence McEvoy, John McLauchlan, Soeren Metelmann, Nahida S. Miah, Joanna Middleton, Joyce Mitchell, Ellen G. Murphy, Rebekah Penrice-Randal, Jack Pilgrim, Tessa Prince, P. Matthew Ridley, Debby Sales, Rebecca K. Shears, Benjamin Small, Krishanthi S. Subramaniam, Agnieska Szemiel, Aislynn Taggart, Jolanta Tanianis-Hughes, Jordan Thomas, Erwan Trochu, Libby van Tonder, Eve Wilcock & J. Eunice Zhang
University College London, London, UK
Shahab Aslani, Amita Banerjee, R. Batterham, Gabrielle Baxter, Robert Bell, Anthony David, Emma Denneny, Alun Hughes, W. Lilaonitkul, P. Mehta, Ashkan Pakzad, Bojidar Rangelov, B. Williams, James Willoughby & Moucheng Xu
Hull University Teaching Hospitals NHS Trust and University of Hull, Hull, UK
Paul Atkin, K. Brindle, Michael Crooks, Katie Drury, Nicholas Easom, Rachel Flockton, L. Holdsworth, A. Richards, D. L. Sykes, Susannah Thackray-Nocera & C. Wright
East Kent Hospitals University NHS Foundation Trust, Canterbury, UK
Liam Austin, Eva Beranova, Tracey Cosier, Joanne Deery, Tracy Hazelton, Carly Price, Hazel Ramos, Reanne Solly, Sharon Turney & Heather Weston
Baillie Gifford Pandemic Science Hub, Centre for Inflammation Research, The Queen’s Medical Research Institute, University of Edinburgh, Edinburgh, UK
Nikos Avramidis, J. Kenneth Baillie, Erola Pairo-Castineira & Konrad Rawlik
Roslin Institute, University of Edinburgh, Edinburgh, UK
Nikos Avramidis, J. Kenneth Baillie & Erola Pairo-Castineira
Newcastle upon Tyne Hospitals NHS Foundation Trust and University of Newcastle, Newcastle upon Tyne, UK
A. Ayoub, J. Brown, G. Burns, Gareth Davies, Anthony De Soyza, Carlos Echevarria, Helen Fisher, C. Francis, Alan Greenhalgh, Philip Hogarth, Joan Hughes, Kasim Jiwa, G. Jones, G. MacGowan, D. Price, Avan Sayer, John Simpson, H. Tedd, S. Thomas, Sophie West, M. Witham, S. Wright & A. Young
East Cheshire NHS Trust, Macclesfield, UK
Marta Babores, Maureen Holland, Natalie Keenan, Sharlene Shashaa & Helen Wassall
Sheffield Teaching NHS Foundation Trust and University of Sheffield, Sheffield, UK
J. Bagshaw, M. Begum, K. Birchall, Robyn Butcher, H. Carborn, Flora Chan, Kerry Chapman, Yutung Cheng, Luke Chetham, Cameron Clark, Zach Coburn, Joby Cole, Myles Dixon, Alexandra Fairman, J. Finnigan, H. Foot, David Foote, Amber Ford, Rebecca Gregory, Kate Harrington, L. Haslam, L. Hesselden, J. Hockridge, Ailsa Holbourn, B. Holroyd-Hind, L. Holt, Alice Howell, E. Hurditch, F. Ilyas, Claire Jarman, Allan Lawrie, Ju Hee Lee, Elvina Lee, Rebecca Lenagh, Alison Lye, Irene Macharia, M. Marshall, Angeline Mbuyisa, J. McNeill, Sharon Megson, J. Meiring, L. Milner, S. Misra, Helen Newell, Tom Newman, C. Norman, Lorenza Nwafor, Dibya Pattenadk, Megan Plowright, Julie Porter, Phillip Ravencroft, C. Roddis, J. Rodger, Peter Saunders, J. Sidebottom, Jacqui Smith, Laurie Smith, N. Steele, G. Stephens, R. Stimpson, B. Thamu, N. Tinker, Kim Turner, Helena Turton, Phillip Wade, S. Walker, James Watson, Imogen Wilson & Amira Zawia
University of Nottingham, Nottingham, UK
David Baguley, Chris Coleman, E. Cox, Laura Fabbri, Susan Francis, Ian Hall, E. Hufton, Simon Johnson, Fasih Khan, Paaig Kitterick, Richard Morriss, Nick Selby, Iain Stewart & Louise Wright
Wirral University Teaching Hospital, Wirral, UK
Elisabeth Bailey, Anne Reddington & Andrew Wight
MRC Human Genetics Unit, Institute of Genetics and Cancer, University of Edinburgh, Western General Hospital, Edinburgh, UK
University of Swansea, Swansea, UK
University of Southampton, London, UK
David Baldwin, P. C. Calder, Nathan Huneke & Gemma Simons
Royal Brompton and Harefield Clinical Group, Guy’s and St Thomas’ NHS Foundation Trust, London, UK
R. E. Barker, Daniele Cristiano, N. Dormand, P. George, Mahitha Gummadi, S. Kon, Kamal Liyanage, C. M. Nolan, B. Patel, Suhani Patel, Oliver Polgar, L. Price, P. Shah, Suver Singh & J. A. Walsh
York and Scarborough NHS Foundation Trust, York, UK
Laura Barman, Claire Brookes, K. Elliott, L. Griffiths, Zoe Guy, Kate Howard, Diana Ionita, Heidi Redfearn, Carol Sarginson & Alison Turnbull
NHS Highland, Inverness, UK
Fiona Barrett, A. Donaldson & Beth Sage
Royal Papworth Hospital NHS Foundation Trust, Cambridge, UK
Helen Baxendale, Lucie Garner, C. Johnson, J. Mackie, Alice Michael, J. Newman, Jamie Pack, K. Paques, H. Parfrey, J. Parmar & A. Reddy
University Hospitals of Derby and Burton, Derby, UK
Paul Beckett, Caroline Dickens & Uttam Nanda
NHS Lanarkshire, Hamilton, UK
Murdina Bell, Angela Brown, M. Brown, R. Hamil, Karen Leitch, L. Macliver, Manish Patel, Jackie Quigley, Andrew Smith & B. Welsh
Cambridge University Hospitals NHS Foundation Trust, NIHR Cambridge Clinical Research Facility and University of Cambridge, Cambridge, UK
Areti Bermperi, Isabel Cruz, K. Dempsey, Anne Elmer, Jonathon Fuld, H. Jones, Sherly Jose, Stefan Marciniak, M. Parkes, Carla Ribeiro, Jessica Taylor, Mark Toshner, L. Watson & J. Worsley
Loughborough University, Loughborough, UK
Lettie Bishop & David Stensel
Betsi Cadwallader University Health Board, Bangor, UK
Annette Bolger, Ffyon Davies, Ahmed Haggar, Joanne Lewis, Arwel Lloyd, R. Manley, Emma McIvor, Daniel Menzies, K. Roberts, W. Saxon, David Southern, Christian Subbe & Victoria Whitehead
Nottingham University Hospitals NHS Trust and University of Nottingham, Nottingham, UK
Charlotte Bolton, J. Bonnington, Melanie Chrystal, Catherine Dupont, Paul Greenhaff, Ayushman Gupta, W. Jang, S. Linford, Laura Matthews, Athanasios Nikolaidis, Sabrina Prosper & Andrew Thomas
King’s College London, London, UK
Kate Bramham, M. Brown, Khalida Ismail, Tim Nicholson, Carmen Pariante, Claire Sharpe, Simon Wessely & J. Whitney
Bradford Teaching Hospitals NHS Foundation Trust, Bradford, UK
Lucy Brear, Karen Regan, Dinesh Saralaya & Kim Storton
South London and Maudsley NHS Foundation Trust and King’s College London, London, UK
G. Breen & M. Hotopf
London School of Hygiene and Tropical Medicine, London, UK
Andrew Briggs
Whittington Health NHS Trust, London, UK
E. Bright, P. Crisp, Ruvini Dharmagunawardena & M. Stern
Cardiff and Vale University Health Board, Cardiff, UK
Lauren Broad, Teriann Evans, Matthew Haynes, L. Jones, Lucy Knibbs, Alison McQueen, Catherine Oliver, Kerry Paradowski, Ramsey Sabit & Jenny Williams
Yeovil District Hospital NHS Foundation Trust, Yeovil, UK
Andrew Broadley
University of Birmingham, Birmingham, UK
Mattew Broome, Paul McArdle, Paul Moss, David Thickett, Rachel Upthegrove, Dan Wilkinson, David Wraith & Erin L. Aldera
BHF Centre for Cardiovascular Science, University of Edinburgh, Edinburgh, UK
Anda Bularga
University of Cambridge, Cambridge, UK
Ed Bullmore, Jonathon Heeney, Claudia Langenberg, William Schwaeble, Charlotte Summers & J. Weir McCall
NIHR Leicester Biomedical Research Centre–Respiratory Patient and Public Involvement Group, Leicester, UK
Jenny Bunker, Rhyan Gill & Rashmita Nathu
Imperial College Healthcare NHS Trust and Imperial College London, London, UK
L. Burden, Ellen Calvelo, Bethany Card, Caitlin Carr, Edwin Chilvers, Donna Copeland, P. Cullinan, Patrick Daly, Lynsey Evison, Tamanah Fayzan, Hussain Gordon, Sulaimaan Haq, Gisli Jenkins, Clara King, Onn Min Kon, Katherine March, Myril Mariveles, Laura McLeavey, Silvia Moriera, Unber Munawar, Uchechi Nwanguma, Lorna Orriss-Dib, Alexandra Ross, Maura Roy, Emily Russell, Katherine Samuel, J. Schronce, Neil Simpson, Lawrence Tarusan, David Thomas, Chloe Wood & Najira Yasmin
Harrogate and District NHD Foundation Trust, Harrogate, UK
Tracy Burdett, James Featherstone, Cathy Lawson, Alison Layton, Clare Mills & Lorraine Stephenson
Newcastle University/Chair of NIHR Dementia TRC, Newcastle, UK
Oxford University Hospitals NHS Foundation Trust, Oxford, UK
A. Burns & N. Kanellakis
Tameside and Glossop Integrated Care NHS Foundation Trust, Ashton-under-Lyne, UK
Al-Tahoor Butt, Martina Coulding, Heather Jones, Susan Kilroy, Jacqueline McCormick, Jerome McIntosh, Heather Savill, Victoria Turner & Joanne Vere
University of Oxford, Nuffield Department of Medicine, Oxford, UK
University of Glasgow, Glasgow, UK
Jonathon Cavanagh, S. MacDonald, Kate O’Donnell, John Petrie, Naveed Sattar & Mark Spears
United Lincolnshire Hospitals NHS Trust, Grantham, UK
Manish Chablani & Lynn Osborne
Department of Psychological Medicine, Institute of Psychiatry, Psychology and Neuroscience, King’s College London, London, UK
Trudie Chalder
University Hospital of South Manchester NHS Foundation Trust, Manchester, UK
N. Chaudhuri
University Hospital Southampton NHS Foundation Trust and University of Southampton, Southampton, UK
Caroline Childs, R. Djukanovic, S. Fletcher, Matt Harvey, Mark Jones, Elizabeth Marouzet, B. Marshall, Reena Samuel, T. Sass, Tim Wallis & Helen Wheeler
King’s College Hospital/Guy’s and St Thomas’ NHS FT, London, UK
A. Chiribiri & C. O’Brien
Barts Health NHS Trust, London, UK
K. Chong-James, C. David, W. Y. James, Paul Pfeffer & O. Zongo
NHS Lothian and University of Edinburgh, Edinburgh, UK
Gaunab Choudhury, S. Clohisey, Andrew Deans, J. Furniss, Ewen Harrison, S. Kelly & Aziz Sheikh
School of Cardiovascular Medicine and Sciences. King’s College London, London, UK
Phillip Chowienczyk
Lewisham and Greenwich NHS Trust, London, UK
Hywel Dda University Health Board, Haverfordwest, UK
S. Coetzee, Kim Davies, Rachel Ann Hughes, Ronda Loosley, Heather McGuinness, Abdelrahman Mohamed, Linda O’Brien, Zohra Omar, Emma Perkins, Janet Phipps, Gavin Ross, Abigail Taylor, Helen Tench & Rebecca Wolf-Roberts
NHS Tayside and University of Dundee, Dundee, UK
David Connell, C. Deas, Anne Elliott, J. George, S. Mohammed, J. Rowland, A. R. Solstice, Debbie Sutherland & Caroline Tee
Swansea Bay University Health Board, Port Talbot, UK
Lynda Connor, Amanda Cook, Gwyneth Davies, Tabitha Rees, Favas Thaivalappil & Caradog Thomas
Faculty of Medicine, Nursing and Health Sciences, School of Biomedical Sciences, Monash University, Melbourne, Victoria, Australia
Eamon Coughlan
Rotherham NHS Foundation Trust, Rotherham, UK
Alison Daniels, Anil Hormis, Julie Ingham & Lisa Zeidan
Salford Royal NHS Foundation Trust, Salford, UK
P. Dark, Nawar Diar-Bakerly, D. Evans, E. Hardy, Alice Harvey, D. Holgate, Sean Knight, N. Mairs, N. Majeed, L. McMorrow, J. Oxton, Jessica Pendlebury, C. Summersgill, R. Ugwuoke & S. Whittaker
Cwm Taf Morgannwg University Health Board, Mountain Ash, UK
Ellie Davies, Cerys Evenden, Alyson Hancock, Kia Hancock, Ceri Lynch, Meryl Rees, Lisa Roche, Natalie Stroud & T. Thomas-Woods
Borders General Hospital, NHS Borders, Melrose, UK
Joy Dawson, Hosni El-Taweel & Leanne Robinson
Aneurin Bevan University Health Board, Caerleon, UK
Amanda Dell, Sara Fairbairn, Nancy Hawkings, Jill Haworth, Michaela Hoare, Victoria Lewis, Alice Lucey, Georgia Mallison, Heeah Nassa, Chris Pennington, Andrea Price, Claire Price, Andrew Storrie, Gemma Willis & Susan Young
University of Exeter Medical School, Exeter, UK
London North West University Healthcare NHS Trust, London, UK
Shalin Diwanji, Sambasivarao Gurram, Padmasayee Papineni, Sheena Quaid, Gerlynn Tiongson & Ekaterina Watson
Alzheimer’s Research UK, Cambridge, UK
Hannah Dobson
Health and Care Research Wales, Cardiff, UK
Yvette Ellis
University of Bristol, Bristol, UK
Jonathon Evans
University of Sheffield, Sheffield, UK
L. Finnigan, Laura Saunders & James Wild
Great Western Hospital Foundation Trust, Swindon, UK
Eva Fraile & Jacinta Ugoji
Royal Devon and Exeter NHS Trust, Barnstaple, UK
Michael Gibbons
Kettering General Hospital NHS Trust, Kettering, UK
Anne-Marie Guerdette, Melanie Hewitt, R. Reddy, Katie Warwick & Sonia White
NIHR Leicester Biomedical Research Centre, Leicester, UK
Beatriz Guillen-Guio
University of Leeds, Leeds, UK
Elspeth Guthrie & Max Henderson
Royal Surrey NHS Foundation Trust, Cranleigh, UK
Mark Halling-Brown & Katherine McCullough
Chesterfield Royal Hospital NHS Trust, Calow, UK
Edward Harris & Claire Sampson
Long Covid Support, London, UK
Claire Hastie, Natalie Rogers & Nikki Smith
King’s College Hospital, NHS Foundation Trust and King’s College London, London, UK
Department of Oncology and Metabolism, University of Sheffield, Sheffield, UK
Simon Heller
NIHR Office for Clinical Research Infrastructure, London, UK
Katie Holmes
Asthma UK and British Lung Foundation Partnership, London, UK
Ian Jarrold & Samantha Walker
North Middlesex University Hospital NHS Trust, London, UK
Bhagy Jayaraman & Tessa Light
Action for Pulmonary Fibrosis, Peterborough, UK
Cardiff University, National Centre for Mental Health, Cardiff, UK
McPin Foundation, London, UK
Thomas Kabir
Roslin Institute, The University of Edinburgh, Edinburgh, UK
Steven Kerr
The Hillingdon Hospitals NHS Foundation Trust, London, UK
Samantha Kon, G. Landers, Harpreet Lota, Mariam Nasseri & Sofiya Portukhay
Queen Mary University of London, London, UK
Ania Korszun
Swansea University, Swansea Welsh Network, Hywel Dda University Health Board, Swansea, UK
Royal Infirmary of Edinburgh, NHS Lothian, Edinburgh, UK
Nazir I. Lone
Barts Heart Centre, London, UK
Barts Health NHS Trust and Queen Mary University of London, London, UK
Adrian Martineau
Salisbury NHS Foundation Trust, Salisbury, UK
Wadzanai Matimba-Mupaya & Sophia Strong-Sheldrake
University of Newcastle, Newcastle, UK
Hamish McAllister-Williams, Stella-Maria Paddick, Anthony Rostron & John Paul Taylor
Gateshead NHS Trust, Gateshead, UK
W. McCormick, Lorraine Pearce, S. Pugmire, Wendy Stoker & Ann Wilson
Manchester Centre for Clinical Neurosciences, Salford Royal NHS Foundation Trust, Manchester, UK
Katherine McIvor
Kidney Research UK, Peterborough, UK
Aisling McMahon
NHS Dumfries and Galloway, Dumfries, UK
Michael McMahon & Paula Neill
Swansea University, Swansea, UK
MQ Mental Health Research, London, UK
Lea Milligan
BHF Centre for Cardiovascular Science, Usher Institute of Population Health Sciences and Informatics, University of Edinburgh, Edinburgh, UK
Nicholas Mills
Shropshire Community Health NHS Trust, Shropshire, UK
Sharon Painter, Johanne Tomlinson & Louise Warburton
Somerset NHS Foundation Trust, Taunton, UK
Sue Palmer, Dawn Redwood, Jo Tilley, Carinna Vickers & Tania Wainwright
Francis Crick Institute, London, UK
Markus Ralser
Manchester University NHD Foundation Trust, Manchester, UK
Pilar Rivera-Ortega
Diabetes UK, University of Glasgow, Glasgow, UK
Elizabeth Robertson
Barnsley Hospital NHS Foundation Trust, Barnsley, UK
Amy Sanderson
MRC–University of Glasgow Centre for Virus Research, Glasgow, UK
Janet Scott
Diabetes UK, London, UK
Kamini Shah
British Heart Foundation Centre, King’s College London, London, UK
King’s College Hospital NHS Foundation Trust, London, UK
University Hospitals Birmingham NHS Foundation Trust and University of Birmingham, Birmingham, UK
Institute of Cardiovascular and Medical Sciences, BHF Glasgow Cardiovascular Research Centre, University of Glasgow, Glasgow, UK
University College London NHS Foundation Trust, London and Barts Health NHS Trust, London, UK
Northumbria University, Newcastle upon Tyne, UK
Ioannis Vogiatzis
Swansea University and Swansea Welsh Network, Swansea, UK
N. Williams
DUK | NHS Digital, Salford Royal Foundation Trust, Salford, UK
Queen Alexandra Hospital, Portsmouth, UK
- Kayode Adeniji
Princess Royal Hospital, Haywards Heath, UK
Daniel Agranoff & Chi Eziefula
Bassetlaw Hospital, Bassetlaw, UK
Darent Valley Hospital, Dartford, UK
Queen Elizabeth the Queen Mother Hospital, Margate, UK
Ana Alegria
School of Informatics, University of Edinburgh, Edinburgh, UK
Beatrice Alex, Benjamin Bach & James Scott-Brown
North East and North Cumbria Ingerated, Newcastle upon Tyne, UK
Section of Biomolecular Medicine, Division of Systems Medicine, Department of Metabolism, Digestion and Reproduction, Imperial College London, London, UK
Petros Andrikopoulos, Kanta Chechi, Marc-Emmanuel Dumas, Julian Griffin, Sonia Liggi & Zoltan Takats
Section of Genomic and Environmental Medicine, Respiratory Division, National Heart and Lung Institute, Imperial College London, London, UK
Petros Andrikopoulos, Marc-Emmanuel Dumas, Michael Olanipekun & Anthonia Osagie
John Radcliffe Hospital, Oxford, UK
Brian Angus
Royal Albert Edward Infirmary, Wigan, UK
Abdul Ashish
Manchester Royal Infirmary, Manchester, UK
Dougal Atkinson
MRC Human Genetics Unit, Institute of Genetics and Cancer, University of Edinburgh, Western General Hospital, Crewe Road, Edinburgh, UK
Section of Molecular Virology, Imperial College London, London, UK
Wendy S. Barclay
Furness General Hospital, Barrow-in-Furness, UK
Shahedal Bari
Hull University Teaching Hospital Trust, Kingston upon Hull, UK
Gavin Barlow
Hillingdon Hospital, Hillingdon, UK
Stella Barnass
St Thomas’ Hospital, London, UK
Nicholas Barrett
Coventry and Warwickshire, Coventry, UK
Christopher Bassford
St Michael’s Hospital, Bristol, UK
Sneha Basude
Stepping Hill Hospital, Stockport, UK
David Baxter
Royal Liverpool University Hospital, Liverpool, UK
Michael Beadsworth
Bristol Royal Hospital Children’s, Bristol, UK
Jolanta Bernatoniene
Scarborough Hospital, Scarborough, UK
John Berridge
Golden Jubilee National Hospital, Clydebank, UK
Colin Berry
Liverpool Heart and Chest Hospital, Liverpool, UK
Nicola Best
Centre for Inflammation Research, The Queen’s Medical Research Institute, University of Edinburgh, Edinburgh, UK
Debby Bogaert & Clark D. Russell
James Paget University Hospital, Great Yarmouth, UK
Pieter Bothma & Darell Tupper-Carey
Aberdeen Royal Infirmary, Aberdeen, UK
Robin Brittain-Long
Adamson Hospital, Cupar, UK
Naomi Bulteel
Royal Devon and Exeter Hospital, Exeter, UK
Worcestershire Royal Hospital, Worcester, UK
Andrew Burtenshaw
ISARIC Global Support Centre, Centre for Tropical Medicine and Global Health, Nuffield Department of Medicine, University of Oxford, Oxford, UK
Gail Carson, Laura Merson & Louise Sigfrid
Conquest Hospital, Hastings, UK
Vikki Caruth
The James Cook University Hospital, Middlesbrough, UK
David Chadwick
Dorset County Hospital, Dorchester, UK
Duncan Chambler
Antimicrobial Resistance and Hospital Acquired Infection Department, Public Health England, London, UK
Meera Chand
Department of Epidemiology and Biostatistics, School of Public Health, Faculty of Medicine, Imperial College London, London, UK
Kanta Chechi
Royal Bournemouth General Hospital, Bournemouth, UK
Harrogate Hospital, Harrogate, UK
Jenny Child
Royal Blackburn Teaching Hospital, Blackburn, UK
Srikanth Chukkambotla
Edinburgh Clinical Research Facility, University of Edinburgh, Edinburgh, UK
Richard Clark, Audrey Coutts, Lorna Donelly, Angie Fawkes, Tammy Gilchrist, Katarzyna Hafezi, Louise MacGillivray, Alan Maclean, Sarah McCafferty, Kirstie Morrice, Lee Murphy & Nicola Wrobel
Torbay Hospital, Torquay, UK
Northern General Hospital, Sheffield, UK
Paul Collini, Cariad Evans & Gary Mills
Liverpool Clinical Trials Centre, University of Liverpool, Liverpool, UK
Marie Connor, Jo Dalton, Chloe Donohue, Carrol Gamble, Michelle Girvan, Sophie Halpin, Janet Harrison, Clare Jackson, Laura Marsh, Stephanie Roberts & Egle Saviciute
Department of Infectious Disease, Imperial College London, London, UK
Graham S. Cooke & Shiranee Sriskandan
St Georges Hospital (Tooting), London, UK
Catherine Cosgrove
Blackpool Victoria Hospital, Blackpool, UK
Jason Cupitt & Joanne Howard
The Royal London Hospital, London, UK
Maria-Teresa Cutino-Moguel
MRC-University of Glasgow Centre for Virus Research, Glasgow, UK
Ana da Silva Filipe, Antonia Y. W. Ho, Sarah E. McDonald, Massimo Palmarini, David L. Robertson, Janet T. Scott & Emma C. Thomson
Salford Royal Hospital, Salford, UK
University Hospital of North Durham, Durham, UK
Chris Dawson
Norfolk and Norwich University Hospital, Norwich, UK
Samir Dervisevic
Intensive Care Unit, Royal Infirmary Edinburgh, Edinburgh, UK
Annemarie B. Docherty & Seán Keating
Institute of Infection, Veterinary and Ecological Sciences, Faculty of Health and Life Sciences, University of Liverpool, Liverpool, UK
Cara Donegan & Rebecca G. Spencer
Salisbury District Hospital, Salisbury, UK
Phil Donnison
National Phenome Centre, Department of Metabolism, Digestion and Reproduction, Imperial College London, London, UK
Gonçalo dos Santos Correia, Matthew Lewis, Lynn Maslen, Caroline Sands, Zoltan Takats & Panteleimon Takis
Section of Bioanalytical Chemistry, Department of Metabolism, Digestion and Reproduction, Imperial College London, London, UK
Gonçalo dos Santos Correia, Matthew Lewis, Lynn Maslen, Caroline Sands & Panteleimon Takis
Guy’s and St Thomas’, NHS Foundation Trust, London, UK
Sam Douthwaite, Michael MacMahon, Marlies Ostermann & Manu Shankar-Hari
The Royal Oldham Hospital, Oldham, UK
Andrew Drummond
European Genomic Institute for Diabetes, Institut Pasteur de Lille, Lille University Hospital, University of Lille, Lille, France
Marc-Emmanuel Dumas
McGill University and Genome Quebec Innovation Centre, Montreal, Qeubec, Canada
National Infection Service, Public Health England, London, UK
Jake Dunning & Maria Zambon
Hereford Count Hospital, Hereford, UK
Ingrid DuRand
Southampton General Hospital, Southampton, UK
Ahilanadan Dushianthan
Northampton General Hospital, Northampton, UK
Tristan Dyer
University Hospital of Wales, Cardiff, UK
Chrisopher Fegan
University Hospitals Bristol NHS Foundation Trust, Bristol, UK
Liverpool School of Tropical Medicine, Liverpool, UK
Tom Fletcher
Leighton Hospital, Crewe, UK
Duncan Fullerton & Elijah Matovu
Manor Hospital, Walsall, UK
Scunthorpe Hospital, Scunthorpe, UK
Sanjeev Garg
Cambridge University Hospital, Cambridge, UK
Effrossyni Gkrania-Klotsas
West Suffolk NHS Foundation Trust, Bury St Edmunds, UK
Basingstoke and North Hampshire Hospital, Basingstoke, UK
Arthur Goldsmith
North Cumberland Infirmary, Carlisle, UK
Clive Graham
Paediatric Liver, GI and Nutrition Centre and MowatLabs, King’s College Hospital, London, UK
Tassos Grammatikopoulos
Institute of Liver Studies, King’s College London, London, UK
Institute of Microbiology and Infection, University of Birmingham, Birmingham, UK
Christopher A. Green
Department of Molecular and Clinical Cancer Medicine, University of Liverpool, Liverpool, UK
William Greenhalf
Institute for Global Health, University College London, London, UK
Rishi K. Gupta
NIHR Health Protection Research Unit, Institute of Infection, Veterinary and Ecological Sciences, Faculty of Health and Life Sciences, University of Liverpool, Liverpool, UK
Hayley Hardwick, Malcolm G. Semple, Tom Solomon & Lance C. W. Turtle
Warwick Hospital, Warwick, UK
Elaine Hardy
Birmingham Children’s Hospital, Birmingham, UK
Stuart Hartshorn
Nottingham City Hospital, Nottingham, UK
Daniel Harvey
Glangwili Hospital Child Health Section, Carmarthen, UK
Peter Havalda
Alder Hey Children’s Hospital, Liverpool, UK
Daniel B. Hawcutt
Department of Infectious Diseases, Queen Elizabeth University Hospital, Glasgow, UK
Antonia Y. W. Ho
Bronglais General Hospital, Aberystwyth, UK
Maria Hobrok
Worthing Hospital, Worthing, UK
Luke Hodgson
Centre for Tropical Medicine and Global Health, Nuffield Department of Medicine, University of Oxford, Oxford, UK
Peter W. Horby
Rotheram District General Hospital, Rotheram, UK
Anil Hormis
Virology Reference Department, National Infection Service, Public Health England, Colindale Avenue, London, UK
Samreen Ijaz
Royal Free Hospital, London, UK
Michael Jacobs & Padmasayee Papineni
Homerton Hospital, London, UK
Airedale Hospital, Airedale, UK
Paul Jennings
Basildon Hospital, Basildon, UK
Agilan Kaliappan
The Christie NHS Foundation Trust, Manchester, UK
Vidya Kasipandian
University Hospital Lewisham, London, UK
Stephen Kegg
The Whittington Hospital, London, UK
Michael Kelsey
Southmead Hospital, Bristol, UK
Jason Kendall
Sheffield Childrens Hospital, Sheffield, UK
Caroline Kerrison
Royal United Hospital, Bath, UK
Ian Kerslake
Department of Pharmacology, University of Liverpool, Liverpool, UK
Nuffield Department of Medicine, Peter Medawar Building for Pathogen Research, University of Oxford, Oxford, UK
Paul Klenerman
Translational Gastroenterology Unit, Nuffield Department of Medicine, University of Oxford, Oxford, UK
Public Health Scotland, Edinburgh, UK
Susan Knight, Eva Lahnsteiner & Sarah Tait
Western General Hospital, Edinburgh, UK
Oliver Koch
Southend University Hospital NHS Foundation Trust, Southend-on-Sea, UK
Gouri Koduri
Hinchingbrooke Hospital, Huntingdon, UK
George Koshy & Tamas Leiner
Royal Preston Hospital, Fulwood, UK
Shondipon Laha
University Hospital (Coventry), Coventry, UK
Steven Laird
The Walton Centre, Liverpool, UK
Susan Larkin
ISARIC, Global Support Centre, COVID-19 Clinical Research Resources, Epidemic diseases Research Group, Oxford (ERGO), University of Oxford, Oxford, UK
James Lee & Daniel Plotkin
Centre for Health Informatics, Division of Informatics, Imaging and Data Science, School of Health Sciences, Faculty of Biology, Medicine and Health, University of Manchester, Manchester Academic Health Science Centre, Manchester, UK
Gary Leeming
Hull Royal Infirmary, Hull, UK
Patrick Lillie
Nottingham University Hospitals NHS Trust:, Nottingham, UK
Wei Shen Lim
Darlington Memorial Hospital, Darlington, UK
Queen Elizabeth Hospital (Gateshead), Gateshead, UK
Vanessa Linnett
Warrington Hospital, Warrington, UK
Jeff Little
Bristol Royal Hospital for Children, Bristol, UK
Mark Lyttle
St Mary’s Hospital (Isle of Wight), Isle of Wight, UK
Emily MacNaughton
The Tunbridge Wells Hospital, Royal Tunbridge Wells, UK
Ravish Mankregod
Huddersfield Royal, Huddersfield, UK
Countess of Chester Hospital, Liverpool, UK
Ruth McEwen & Lawrence Wilson
Frimley Park Hospital, Frimley, UK
Manjula Meda
Nuffield Department of Medicine, John Radcliffe Hospital, Oxford, UK
Alexander J. Mentzer
Department of Microbiology/Infectious Diseases, Oxford University Hospitals NHS Foundation Trust, John Radcliffe Hospital, Oxford, UK
MRC Human Genetics Unit, MRC Institute of Genetics and Molecular Medicine, University of Edinburgh, Edinburgh, UK
Alison M. Meynert & Murray Wham
St James University Hospital, Leeds, UK
Jane Minton
Arrowe Park Hospital, Birkenhead, UK
Kavya Mohandas
Great Ormond Street Hospital, London, UK
Royal Shrewsbury Hospital, Shrewsbury, UK
Addenbrookes Hospital, Cambridge, UK
Elinoor Moore
Institute of Infection, Veterinary and Ecological Sciences, University of Liverpool, Liverpool, UK
Shona C. Moore, William A. Paxton & Georgios Pollakis
East Surrey Hospital (Redhill), Redhill, UK
Patrick Morgan
Burton Hospital, Burton, UK
Craig Morris & Tim Reynolds
Peterborough City Hospital, Peterborough, UK
Katherine Mortimore
Kent and Canterbury Hospital, Canterbury, UK
Samuel Moses
Weston Area General Trust, Bristol, UK
Mbiye Mpenge
Bedfordshire Hospital, Bedfordshire, UK
Rohinton Mulla
Glasgow Royal Infirmary, Glasgow, UK
Michael Murphy
Macclesfield General Hospital, Macclesfield, UK
Thapas Nagarajan
Derbyshire Healthcare, Derbyshire, UK
Megan Nagel
Chelsea and Westminster Hospital, London, UK
Mark Nelson & Matthew K. O’Shea
Watford General Hospital, Watford, UK
Lillian Norris & Tom Stambach
EPCC, University of Edinburgh, Edinburgh, UK
Lucy Norris
Section of Biomolecular Medicine, Division of Systems Medicine, Department of Metabolism, Digestion and Reproduction, London, UK
Michael Olanipekun
Imperial College Healthcare NHS Trust: London, London, UK
Peter J. M. Openshaw
Division of Systems Medicine, Department of Metabolism, Digestion and Reproduction, Imperial College London, London, UK
Anthonia Osagie
Prince Philip Hospital, Llanelli, UK
Igor Otahal & Andrew Workman
George Eliot Hospital – Acute Services, Nuneaton, UK
Molecular and Clinical Cancer Medicine, Institute of Systems, Molecular and Integrative Biology, University of Liverpool, Liverpool, UK
Carlo Palmieri
Clatterbridge Cancer Centre NHS Foundation Trust, Liverpool, UK
Kettering General Hospital, Kettering, UK
Selva Panchatsharam
University Hospitals of North Midlands NHS Trust, North Midlands, UK
Danai Papakonstantinou
Russells Hall Hospital, Dudley, UK
Hassan Paraiso
Harefield Hospital, Harefield, UK
Lister Hospital, Lister, UK
Natalie Pattison
Musgrove Park Hospital, Taunton, UK
Justin Pepperell
Kingston Hospital, Kingston, UK
Mark Peters
Queen’s Hospital, Romford, UK
Mandeep Phull
Southport and Formby District General Hospital, Southport, UK
Stefania Pintus
St George’s University of London, London, UK
Tim Planche
King’s College Hospital (Denmark Hill), London, UK
Centre for Clinical Infection and Diagnostics Research, Department of Infectious Diseases, School of Immunology and Microbial Sciences, King’s College London, London, UK
Nicholas Price
Department of Infectious Diseases, Guy’s and St Thomas’ NHS Foundation Trust, London, UK
The Clatterbridge Cancer Centre NHS Foundation, Bebington, UK
David Price
The Great Western Hospital, Swindon, UK
Rachel Prout
Ninewells Hospital, Dundee, UK
Nikolas Rae
Institute of Evolutionary Biology, University of Edinburgh, Edinburgh, UK
Andrew Rambaut
Poole Hospital NHS Trust, Poole, UK
Henrik Reschreiter
William Harvey Hospital, Ashford, UK
Neil Richardson
King’s Mill Hospital, Sutton-in-Ashfield, UK
Mark Roberts
Liverpool Women’s Hospital, Liverpool, UK
Devender Roberts
Pinderfields Hospital, Wakefield, UK
Alistair Rose
North Devon District Hospital, Barnstaple, UK
Guy Rousseau
Queen Elizabeth Hospital, Birmingham, UK
Tameside General Hospital, Ashton-under-Lyne, UK
Brendan Ryan
City Hospital (Birmingham), Birmingham, UK
Taranprit Saluja
Department of Pediatrics and Virology, St Mary’s Medical School Bldg, Imperial College London, London, UK
Vanessa Sancho-Shimizu
The Newcastle Upon Tyne Hospitals NHS Foundation Trust, Newcastle Upon Tyne, UK
Matthias Schmid
NHS Greater Glasgow and Clyde, Glasgow, UK
Janet T. Scott
Respiratory Medicine, Institute in The Park, University of Liverpool, Alder Hey Children’s Hospital, Liverpool, UK
Malcolm G. Semple
Broomfield Hospital, Broomfield, UK
Stoke Mandeville, UK
Prad Shanmuga
University Hospital of North Tees, Stockton-on-Tees, UK
Anil Sharma
Institute of Translational Medicine, University of, Liverpool, Merseyside, UK
Victoria E. Shaw
Royal Manchester Children’s Hospital, Manchester, UK
Anna Shawcross
New Cross Hospital, Wolverhampton, UK
Jagtur Singh Pooni
Bedford Hospital, Bedford, UK
Jeremy Sizer
Colchester General Hospital, Colchester, UK
Richard Smith
University Hospital Birmingham NHS Foundation Trust, Birmingham, UK
Catherine Snelson & Tony Whitehouse
Walton Centre NHS Foundation Trust, Liverpool, UK
Tom Solomon
Chesterfield Royal Hospital, Calow, UK
Nick Spittle
MRC Centre for Molecular Bacteriology and Infection, Imperial College London, London, UK
Shiranee Sriskandan
Princess Alexandra Hospital, Harlow, UK
Nikki Staines & Shico Visuvanathan
Milton Keynes Hospital, Eaglestone, UK
Richard Stewart
Division of Structural Biology, The Wellcome Centre for Human Genetics, University of Oxford, Oxford, UK
David Stuart
Royal Bolton Hopital, Farnworth, UK
Pradeep Subudhi
Department of Medicine, University of Cambridge, Cambridge, UK
Charlotte Summers
Department of Child Life and Health, University of Edinburgh, Edinburgh, UK
Olivia V. Swann
Royal Gwent (Newport), Newport, UK
Tamas Szakmany
The Royal Marsden Hospital (London), London, UK
Kate Tatham
Blood Borne Virus Unit, Virus Reference Department, National Infection Service, Public Health England, London, UK
Richard S. Tedder
Transfusion Microbiology, National Health Service Blood and Transplant, London, UK
Department of Medicine, Imperial College London, London, UK
Queen Victoria Hospital (East Grinstead), East Grinstead, UK
Leeds Teaching Hospitals NHS Trust, Leeds, UK
Robert Thompson
Royal Stoke University Hospital, Stoke-on-Trent, UK
Chris Thompson
Whiston Hospital, Rainhill, UK
Ascanio Tridente
Tropical and Infectious Disease Unit, Royal Liverpool University Hospital, Liverpool, UK
Lance C. W. Turtle
Croydon University Hospital, Thornton Heath, UK
Mary Twagira
Gloucester Royal, Gloucester, UK
Nick Vallotton
West Hertfordshire Teaching Hospitals NHS Trust, Hertfordshire, UK
Rama Vancheeswaran
North Middlesex Hospital, London, UK
Rachel Vincent
Medway Maritime Hospital, Gillingham, UK
Lisa Vincent-Smith
Royal Papworth Hospital Everard, Cambridge, UK
Alan Vuylsteke
Derriford (Plymouth), Plymouth, UK
St Helier Hospital, Sutton, UK
Rachel Wake
Royal Berkshire Hospital, Reading, UK
Andrew Walden
Royal Liverpool Hospital, Liverpool, UK
Ingeborg Welters
Bradford Royal infirmary, Bradford, UK
Paul Whittaker
Central Middlesex, London, UK
Ashley Whittington
Royal Cornwall Hospital (Tresliske), Truro, UK
Meme Wijesinghe
North Bristol NHS Trust, Bristol, UK
Martin Williams
St. Peter’s Hospital, Runnymede, UK
Stephen Winchester
Leicester Royal Infirmary, Leicester, UK
Martin Wiselka
Grantham and District Hospital, Grantham, UK
Adam Wolverson
Aintree University Hospital, Liverpool, UK
Daniel G. Wootton
North Tyneside General Hospital, North Shields, UK
Bryan Yates
Queen Elizabeth Hospital, King’s Lynn, UK
Peter Young
You can also search for this author in PubMed Google Scholar
PHOSP-COVID collaborative group
- Kathryn Abel
- , H. Adamali
- , Davies Adeloye
- , Oluwaseun Adeyemi
- , Rita Adrego
- , Laura Aguilar Jimenez
- , Shanaz Ahmad
- , N. Ahmad Haider
- , Rubina Ahmed
- , Nyarko Ahwireng
- , Mark Ainsworth
- , Asma Alamoudi
- , Mariam Ali
- , M. Aljaroof
- , Louise Allan
- , Richard Allen
- , Lisa Allerton
- , Lynne Allsop
- , Ann Marie Allt
- , Paula Almeida
- , Bashar Al-Sheklly
- , Danny Altmann
- , Maria Alvarez Corral
- , Shannon Amoils
- , David Anderson
- , Charalambos Antoniades
- , Gill Arbane
- , Ava Maria Arias
- , Cherie Armour
- , Lisa Armstrong
- , Natalie Armstrong
- , David Arnold
- , H. Arnold
- , A. Ashish
- , Andrew Ashworth
- , M. Ashworth
- , Shahab Aslani
- , Hosanna Assefa-Kebede
- , Paul Atkin
- , Catherine Atkin
- , Raminder Aul
- , Hnin Aung
- , Liam Austin
- , Cristina Avram
- , Nikos Avramidis
- , Marta Babores
- , Rhiannon Baggott
- , J. Bagshaw
- , David Baguley
- , Elisabeth Bailey
- , J. Kenneth Baillie
- , Steve Bain
- , Majda Bakali
- , E. Baldry
- , Molly Baldwin
- , David Baldwin
- , Clive Ballard
- , Amita Banerjee
- , Dongchun Bang
- , R. E. Barker
- , Laura Barman
- , Perdita Barran
- , Shaney Barratt
- , Fiona Barrett
- , Donna Basire
- , Neil Basu
- , Michelle Bates
- , R. Batterham
- , Helen Baxendale
- , Gabrielle Baxter
- , Hannah Bayes
- , M. Beadsworth
- , Paul Beckett
- , Paul Beirne
- , Murdina Bell
- , Robert Bell
- , Kaytie Bennett
- , Eva Beranova
- , Areti Bermperi
- , Anthony Berridge
- , Colin Berry
- , Sarah Betts
- , Emily Bevan
- , Kamaldeep Bhui
- , Michelle Bingham
- , K. Birchall
- , Lettie Bishop
- , Karen Bisnauthsing
- , John Blaikely
- , Angela Bloss
- , Annette Bolger
- , Charlotte Bolton
- , J. Bonnington
- , A. Botkai
- , Charlotte Bourne
- , Michelle Bourne
- , Kate Bramham
- , Lucy Brear
- , Jonathon Breeze
- , Katie Breeze
- , Andrew Briggs
- , E. Bright
- , Christopher Brightling
- , Simon Brill
- , K. Brindle
- , Lauren Broad
- , Andrew Broadley
- , Claire Brookes
- , Mattew Broome
- , Vanessa Brown
- , Ammani Brown
- , Angela Brown
- , Jeremy Brown
- , Terry Brugha
- , Nigel Brunskill
- , Phil Buckley
- , Anda Bularga
- , Ed Bullmore
- , Jenny Bunker
- , L. Burden
- , Tracy Burdett
- , David Burn
- , John Busby
- , Robyn Butcher
- , Al-Tahoor Butt
- , P. Cairns
- , P. C. Calder
- , Ellen Calvelo
- , H. Carborn
- , Bethany Card
- , Caitlin Carr
- , Liesel Carr
- , G. Carson
- , Penny Carter
- , Anna Casey
- , M. Cassar
- , Jonathon Cavanagh
- , Manish Chablani
- , Trudie Chalder
- , James D. Chalmers
- , Rachel Chambers
- , Flora Chan
- , K. M. Channon
- , Kerry Chapman
- , Amanda Charalambou
- , N. Chaudhuri
- , A. Checkley
- , Yutung Cheng
- , Luke Chetham
- , Caroline Childs
- , Edwin Chilvers
- , H. Chinoy
- , A. Chiribiri
- , K. Chong-James
- , N. Choudhury
- , Gaunab Choudhury
- , Phillip Chowienczyk
- , C. Christie
- , Melanie Chrystal
- , Cameron Clark
- , David Clark
- , Jude Clarke
- , S. Clohisey
- , G. Coakley
- , Zach Coburn
- , S. Coetzee
- , Joby Cole
- , Chris Coleman
- , Florence Conneh
- , David Connell
- , Bronwen Connolly
- , Lynda Connor
- , Amanda Cook
- , Shirley Cooper
- , B. Cooper
- , Josh Cooper
- , Donna Copeland
- , Tracey Cosier
- , Eamon Coughlan
- , Martina Coulding
- , C. Coupland
- , Thelma Craig
- , Daniele Cristiano
- , Michael Crooks
- , Andy Cross
- , Isabel Cruz
- , P. Cullinan
- , D. Cuthbertson
- , Luke Daines
- , Matthhew Dalton
- , Patrick Daly
- , Alison Daniels
- , Joanne Dasgin
- , Anthony David
- , Ffyon Davies
- , Ellie Davies
- , Kim Davies
- , Gareth Davies
- , Gwyneth Davies
- , Melanie Davies
- , Joy Dawson
- , Camilla Dawson
- , Enya Daynes
- , Anthony De Soyza
- , Bill Deakin
- , Andrew Deans
- , Joanne Deery
- , Sylviane Defres
- , Amanda Dell
- , K. Dempsey
- , Emma Denneny
- , J. Dennis
- , Ruvini Dharmagunawardena
- , Nawar Diar-Bakerly
- , Caroline Dickens
- , A. Dipper
- , Sarah Diver
- , Shalin Diwanji
- , Myles Dixon
- , R. Djukanovic
- , Hannah Dobson
- , S. L. Dobson
- , Annemarie B. Docherty
- , A. Donaldson
- , N. Dormand
- , Andrew Dougherty
- , Rachael Dowling
- , Stephen Drain
- , Katharine Draxlbauer
- , Katie Drury
- , Pearl Dulawan
- , A. Dunleavy
- , Sarah Dunn
- , Catherine Dupont
- , Joanne Earley
- , Nicholas Easom
- , Carlos Echevarria
- , Sarah Edwards
- , C. Edwardson
- , Claudia Efstathiou
- , Anne Elliott
- , K. Elliott
- , Yvette Ellis
- , Anne Elmer
- , Omer Elneima
- , Hosni El-Taweel
- , Teriann Evans
- , Ranuromanana Evans
- , Rachael A. Evans
- , Jonathon Evans
- , Cerys Evenden
- , Lynsey Evison
- , Laura Fabbri
- , Sara Fairbairn
- , Alexandra Fairman
- , K. Fallon
- , David Faluyi
- , Clair Favager
- , Tamanah Fayzan
- , James Featherstone
- , T. Felton
- , V. Ferreira
- , Selina Finney
- , J. Finnigan
- , L. Finnigan
- , Helen Fisher
- , S. Fletcher
- , Rachel Flockton
- , Margaret Flynn
- , David Foote
- , Amber Ford
- , D. Forton
- , Eva Fraile
- , C. Francis
- , Richard Francis
- , Susan Francis
- , Anew Frankel
- , Emily Fraser
- , N. French
- , Jonathon Fuld
- , J. Furniss
- , Lucie Garner
- , N. Gautam
- , John Geddes
- , J. George
- , P. George
- , Michael Gibbons
- , Rhyan Gill
- , Mandy Gill
- , L. Gilmour
- , F. Gleeson
- , Jodie Glossop
- , Sarah Glover
- , Nicola Goodman
- , Camelia Goodwin
- , Bibek Gooptu
- , Hussain Gordon
- , T. Gorsuch
- , M. Greatorex
- , Paul Greenhaff
- , William Greenhalf
- , Alan Greenhalgh
- , Neil J. Greening
- , John Greenwood
- , Rebecca Gregory
- , Heidi Gregory
- , D. Grieve
- , Denise Griffin
- , L. Griffiths
- , Anne-Marie Guerdette
- , Beatriz Guillen-Guio
- , Mahitha Gummadi
- , Ayushman Gupta
- , Sambasivarao Gurram
- , Elspeth Guthrie
- , Kate Hadley
- , Ahmed Haggar
- , Kera Hainey
- , Brigid Hairsine
- , Pranab Haldar
- , Lucy Hall
- , Mark Halling-Brown
- , Alyson Hancock
- , Kia Hancock
- , Neil Hanley
- , Sulaimaan Haq
- , Hayley Hardwick
- , Tim Hardy
- , Beverley Hargadon
- , Kate Harrington
- , Edward Harris
- , Victoria C. Harris
- , Ewen Harrison
- , Paul Harrison
- , Nicholas Hart
- , Alice Harvey
- , Matt Harvey
- , M. Harvie
- , L. Haslam
- , Claire Hastie
- , May Havinden-Williams
- , Jenny Hawkes
- , Nancy Hawkings
- , Jill Haworth
- , A. Hayday
- , Matthew Haynes
- , J. Hazeldine
- , Tracy Hazelton
- , Liam Heaney
- , Cheryl Heeley
- , Jonathon Heeney
- , M. Heightman
- , Simon Heller
- , Max Henderson
- , Helen Henson
- , L. Hesselden
- , Melanie Hewitt
- , Victoria Highett
- , T. Hillman
- , Ling-Pei Ho
- , Michaela Hoare
- , Amy Hoare
- , J. Hockridge
- , Philip Hogarth
- , Ailsa Holbourn
- , Sophie Holden
- , L. Holdsworth
- , D. Holgate
- , Maureen Holland
- , Leah Holloway
- , Katie Holmes
- , Megan Holmes
- , B. Holroyd-Hind
- , Anil Hormis
- , Alexander Horsley
- , Akram Hosseini
- , M. Hotopf
- , Linzy Houchen-Wolloff
- , Luke S. Howard
- , Kate Howard
- , Alice Howell
- , E. Hufton
- , Rachel Ann Hughes
- , Joan Hughes
- , Alun Hughes
- , Amy Humphries
- , Nathan Huneke
- , E. Hurditch
- , John Hurst
- , Masud Husain
- , Tracy Hussell
- , John Hutchinson
- , W. Ibrahim
- , Julie Ingham
- , L. Ingram
- , Diana Ionita
- , Karen Isaacs
- , Khalida Ismail
- , T. Jackson
- , Joseph Jacob
- , W. Y. James
- , Claire Jarman
- , Ian Jarrold
- , Hannah Jarvis
- , Roman Jastrub
- , Bhagy Jayaraman
- , Gisli Jenkins
- , P. Jezzard
- , Kasim Jiwa
- , C. Johnson
- , Simon Johnson
- , Desmond Johnston
- , Caroline Jolley
- , Ian Jones
- , Heather Jones
- , Mark Jones
- , Don Jones
- , Sherly Jose
- , Thomas Kabir
- , G. Kaltsakas
- , Vicky Kamwa
- , N. Kanellakis
- , Sabina Kaprowska
- , Zunaira Kausar
- , Natalie Keenan
- , Steven Kerr
- , Helen Kerslake
- , Angela Key
- , Fasih Khan
- , Kamlesh Khunti
- , Susan Kilroy
- , Bernie King
- , Clara King
- , Lucy Kingham
- , Jill Kirk
- , Paaig Kitterick
- , Paul Klenerman
- , Lucy Knibbs
- , Sean Knight
- , Abigail Knighton
- , Onn Min Kon
- , Samantha Kon
- , Ania Korszun
- , Ivan Koychev
- , Claire Kurasz
- , Prathiba Kurupati
- , Hanan Lamlum
- , G. Landers
- , Claudia Langenberg
- , Lara Lavelle-Langham
- , Allan Lawrie
- , Cathy Lawson
- , Claire Lawson
- , Alison Layton
- , Olivia C. Leavy
- , Ju Hee Lee
- , Elvina Lee
- , Karen Leitch
- , Rebecca Lenagh
- , Victoria Lewis
- , Joanne Lewis
- , Keir Lewis
- , N. Lewis-Burke
- , Felicity Liew
- , Tessa Light
- , Liz Lightstone
- , W. Lilaonitkul
- , S. Linford
- , Anne Lingford-Hughes
- , M. Lipman
- , Kamal Liyanage
- , Arwel Lloyd
- , Nazir I. Lone
- , Ronda Loosley
- , Janet Lord
- , Harpreet Lota
- , Wayne Lovegrove
- , Daniel Lozano-Rojas
- , Alice Lucey
- , Gardiner Lucy
- , E. Lukaschuk
- , Alison Lye
- , Ceri Lynch
- , S. MacDonald
- , G. MacGowan
- , Irene Macharia
- , J. Mackie
- , L. Macliver
- , S. Madathil
- , Gladys Madzamba
- , Nick Magee
- , Murphy Magtoto
- , N. Majeed
- , Flora Malein
- , Georgia Mallison
- , William Man
- , S. Mandal
- , K. Mangion
- , C. Manisty
- , R. Manley
- , Katherine March
- , Stefan Marciniak
- , Philip Marino
- , Myril Mariveles
- , Michael Marks
- , Elizabeth Marouzet
- , Sophie Marsh
- , M. Marshall
- , B. Marshall
- , Jane Martin
- , Adrian Martineau
- , L. M. Martinez
- , Nick Maskell
- , Darwin Matila
- , Wadzanai Matimba-Mupaya
- , Laura Matthews
- , Angeline Mbuyisa
- , Steve McAdoo
- , Hamish McAllister-Williams
- , Paul McArdle
- , Anne McArdle
- , Danny McAulay
- , Hamish J. C. McAuley
- , Gerry McCann
- , W. McCormick
- , Jacqueline McCormick
- , P. McCourt
- , Celeste McCracken
- , Lorcan McGarvey
- , Jade McGinness
- , K. McGlynn
- , Andrew McGovern
- , Heather McGuinness
- , I. B. McInnes
- , Jerome McIntosh
- , Emma McIvor
- , Katherine McIvor
- , Laura McLeavey
- , Aisling McMahon
- , Michael McMahon
- , L. McMorrow
- , Teresa Mcnally
- , M. McNarry
- , J. McNeill
- , Alison McQueen
- , H. McShane
- , Chloe Mears
- , Clare Megson
- , Sharon Megson
- , J. Meiring
- , Lucy Melling
- , Mark Mencias
- , Daniel Menzies
- , Marta Merida Morillas
- , Alice Michael
- , Benedict Michael
- , C. A. Miller
- , Lea Milligan
- , Nicholas Mills
- , Clare Mills
- , George Mills
- , L. Milner
- , Jane Mitchell
- , Abdelrahman Mohamed
- , Noura Mohamed
- , S. Mohammed
- , Philip Molyneaux
- , Will Monteiro
- , Silvia Moriera
- , Anna Morley
- , Leigh Morrison
- , Richard Morriss
- , A. Morrow
- , Paul Moss
- , Alistair Moss
- , K. Motohashi
- , N. Msimanga
- , Elizabeta Mukaetova-Ladinska
- , Unber Munawar
- , Jennifer Murira
- , Uttam Nanda
- , Heeah Nassa
- , Mariam Nasseri
- , Rashmita Nathu
- , Aoife Neal
- , Robert Needham
- , Paula Neill
- , Stefan Neubauer
- , D. E. Newby
- , Helen Newell
- , J. Newman
- , Tom Newman
- , Alex Newton-Cox
- , T. E. Nichols
- , Tim Nicholson
- , Christos Nicolaou
- , Debby Nicoll
- , Athanasios Nikolaidis
- , C. Nikolaidou
- , C. M. Nolan
- , Matthew Noonan
- , C. Norman
- , Petr Novotny
- , Kimon Ntotsis
- , Jose Nunag
- , Lorenza Nwafor
- , Uchechi Nwanguma
- , Joseph Nyaboko
- , Linda O’Brien
- , C. O’Brien
- , Natasha Odell
- , Kate O’Donnell
- , Godwin Ogbole
- , Olaoluwa Olaosebikan
- , Catherine Oliver
- , Zohra Omar
- , Peter J. M. Openshaw
- , D. P. O’Regan
- , Lorna Orriss-Dib
- , Lynn Osborne
- , Rebecca Osbourne
- , Marlies Ostermann
- , Charlotte Overton
- , Jamie Pack
- , Edmund Pacpaco
- , Stella-Maria Paddick
- , Sharon Painter
- , Erola Pairo-Castineira
- , Ashkan Pakzad
- , Sue Palmer
- , Padmasayee Papineni
- , K. Paques
- , Kerry Paradowski
- , Manish Pareek
- , Dhruv Parekh
- , H. Parfrey
- , Carmen Pariante
- , S. Parker
- , M. Parkes
- , J. Parmar
- , Sheetal Patale
- , Manish Patel
- , Suhani Patel
- , Dibya Pattenadk
- , M. Pavlides
- , Sheila Payne
- , Lorraine Pearce
- , John Pearl
- , Dan Peckham
- , Jessica Pendlebury
- , Yanchun Peng
- , Chris Pennington
- , Ida Peralta
- , Emma Perkins
- , Z. Peterkin
- , Tunde Peto
- , Nayia Petousi
- , John Petrie
- , Paul Pfeffer
- , Janet Phipps
- , S. Piechnik
- , John Pimm
- , Karen Piper Hanley
- , Riinu Pius
- , Hannah Plant
- , Tatiana Plekhanova
- , Megan Plowright
- , Krisnah Poinasamy
- , Oliver Polgar
- , Julie Porter
- , Joanna Porter
- , Sofiya Portukhay
- , Natassia Powell
- , A. Prabhu
- , James Pratt
- , Andrea Price
- , Claire Price
- , Carly Price
- , Anne Prickett
- , I. Propescu
- , J. Propescu
- , Sabrina Prosper
- , S. Pugmire
- , Sheena Quaid
- , Jackie Quigley
- , Jennifer K. Quint
- , H. Qureshi
- , I. N. Qureshi
- , K. Radhakrishnan
- , Najib Rahman
- , Markus Ralser
- , Betty Raman
- , Hazel Ramos
- , Albert Ramos
- , Jade Rangeley
- , Bojidar Rangelov
- , Liz Ratcliffe
- , Phillip Ravencroft
- , Konrad Rawlik
- , Anne Reddington
- , Heidi Redfearn
- , Dawn Redwood
- , Annabel Reed
- , Meryl Rees
- , Tabitha Rees
- , Karen Regan
- , Will Reynolds
- , Carla Ribeiro
- , A. Richards
- , Emma Richardson
- , M. Richardson
- , Pilar Rivera-Ortega
- , K. Roberts
- , Elizabeth Robertson
- , Leanne Robinson
- , Emma Robinson
- , Lisa Roche
- , C. Roddis
- , J. Rodger
- , Natalie Rogers
- , Gavin Ross
- , Alexandra Ross
- , Jennifer Rossdale
- , Anthony Rostron
- , Anna Rowe
- , J. Rowland
- , M. J. Rowland
- , A. Rowland
- , Sarah L. Rowland-Jones
- , Maura Roy
- , Igor Rudan
- , Richard Russell
- , Emily Russell
- , Gwen Saalmink
- , Ramsey Sabit
- , Beth Sage
- , T. Samakomva
- , Nilesh Samani
- , A. A. Samat
- , Claire Sampson
- , Katherine Samuel
- , Reena Samuel
- , Z. B. Sanders
- , Amy Sanderson
- , Elizabeth Sapey
- , Dinesh Saralaya
- , Jack Sargant
- , Carol Sarginson
- , Naveed Sattar
- , Kathryn Saunders
- , Peter Saunders
- , Ruth Saunders
- , Laura Saunders
- , Heather Savill
- , Avan Sayer
- , J. Schronce
- , William Schwaeble
- , Janet Scott
- , Kathryn Scott
- , Nick Selby
- , Malcolm G. Semple
- , Marco Sereno
- , Terri Ann Sewell
- , Kamini Shah
- , Ajay Shah
- , Manu Shankar-Hari
- , M. Sharma
- , Claire Sharpe
- , Michael Sharpe
- , Sharlene Shashaa
- , Alison Shaw
- , Victoria Shaw
- , Karen Shaw
- , Aziz Sheikh
- , Sarah Shelton
- , Liz Shenton
- , K. Shevket
- , Aarti Shikotra
- , Sulman Siddique
- , Salman Siddiqui
- , J. Sidebottom
- , Louise Sigfrid
- , Gemma Simons
- , Neil Simpson
- , John Simpson
- , Ananga Singapuri
- , Suver Singh
- , Claire Singh
- , Sally Singh
- , D. Sissons
- , J. Skeemer
- , Katie Slack
- , David Smith
- , Nikki Smith
- , Andrew Smith
- , Jacqui Smith
- , Laurie Smith
- , Susan Smith
- , M. Soares
- , Teresa Solano
- , Reanne Solly
- , A. R. Solstice
- , Tracy Soulsby
- , David Southern
- , D. Sowter
- , Mark Spears
- , Lisa Spencer
- , Fabio Speranza
- , Louise Stadon
- , Stefan Stanel
- , R. Steeds
- , N. Steele
- , Mike Steiner
- , David Stensel
- , G. Stephens
- , Lorraine Stephenson
- , Iain Stewart
- , R. Stimpson
- , Sue Stockdale
- , J. Stockley
- , Wendy Stoker
- , Roisin Stone
- , Will Storrar
- , Andrew Storrie
- , Kim Storton
- , E. Stringer
- , Sophia Strong-Sheldrake
- , Natalie Stroud
- , Christian Subbe
- , Catherine Sudlow
- , Zehra Suleiman
- , Charlotte Summers
- , C. Summersgill
- , Debbie Sutherland
- , D. L. Sykes
- , Nick Talbot
- , Ai Lyn Tan
- , Lawrence Tarusan
- , Vera Tavoukjian
- , Jessica Taylor
- , Abigail Taylor
- , Chris Taylor
- , John Paul Taylor
- , Amelie Te
- , Caroline Tee
- , J. Teixeira
- , Helen Tench
- , Sarah Terry
- , Susannah Thackray-Nocera
- , Favas Thaivalappil
- , David Thickett
- , David Thomas
- , S. Thomas
- , Caradog Thomas
- , Andrew Thomas
- , T. Thomas-Woods
- , A. A. Roger Thompson
- , Tamika Thompson
- , T. Thornton
- , Matthew Thorpe
- , Ryan S. Thwaites
- , Jo Tilley
- , N. Tinker
- , Gerlynn Tiongson
- , Martin Tobin
- , Johanne Tomlinson
- , Mark Toshner
- , T. Treibel
- , K. A. Tripp
- , Drupad Trivedi
- , E. M. Tunnicliffe
- , Alison Turnbull
- , Kim Turner
- , Sarah Turner
- , Victoria Turner
- , E. Turner
- , Sharon Turney
- , Lance Turtle
- , Helena Turton
- , Jacinta Ugoji
- , R. Ugwuoke
- , Rachel Upthegrove
- , Jonathon Valabhji
- , Maximina Ventura
- , Joanne Vere
- , Carinna Vickers
- , Ben Vinson
- , Ioannis Vogiatzis
- , Elaine Wade
- , Phillip Wade
- , Louise V. Wain
- , Tania Wainwright
- , Lilian Wajero
- , Sinead Walder
- , Samantha Walker
- , S. Walker
- , Tim Wallis
- , Sarah Walmsley
- , Simon Walsh
- , J. A. Walsh
- , Louise Warburton
- , T. J. C. Ward
- , Katie Warwick
- , Helen Wassall
- , Samuel Waterson
- , L. Watson
- , Ekaterina Watson
- , James Watson
- , M. Webster
- , J. Weir McCall
- , Carly Welch
- , Simon Wessely
- , Sophie West
- , Heather Weston
- , Helen Wheeler
- , Sonia White
- , Victoria Whitehead
- , J. Whitney
- , S. Whittaker
- , Beverley Whittam
- , V. Whitworth
- , Andrew Wight
- , James Wild
- , Martin Wilkins
- , Dan Wilkinson
- , Nick Williams
- , N. Williams
- , B. Williams
- , Jenny Williams
- , S. A. Williams-Howard
- , Michelle Willicombe
- , Gemma Willis
- , James Willoughby
- , Ann Wilson
- , Imogen Wilson
- , Daisy Wilson
- , Nicola Window
- , M. Witham
- , Rebecca Wolf-Roberts
- , Chloe Wood
- , F. Woodhead
- , Janet Woods
- , Dan Wootton
- , J. Wormleighton
- , J. Worsley
- , David Wraith
- , Caroline Wrey Brown
- , C. Wright
- , S. Wright
- , Louise Wright
- , Inez Wynter
- , Moucheng Xu
- , Najira Yasmin
- , S. Yasmin
- , Tom Yates
- , Kay Por Yip
- , Susan Young
- , Bob Young
- , A. J. Yousuf
- , Amira Zawia
- , Lisa Zeidan
- , Bang Zhao
- , Bang Zheng
- & O. Zongo
- , Daniel Agranoff
- , Ken Agwuh
- , Katie A. Ahmed
- , Dhiraj Ail
- , Erin L. Aldera
- , Ana Alegria
- , Beatrice Alex
- , Sam Allen
- , Petros Andrikopoulos
- , Brian Angus
- , Jane A. Armstrong
- , Abdul Ashish
- , Milton Ashworth
- , Innocent G. Asiimwe
- , Dougal Atkinson
- , Benjamin Bach
- , Siddharth Bakshi
- , Wendy S. Barclay
- , Shahedal Bari
- , Gavin Barlow
- , Samantha L. Barlow
- , Stella Barnass
- , Nicholas Barrett
- , Christopher Bassford
- , Sneha Basude
- , David Baxter
- , Michael Beadsworth
- , Jolanta Bernatoniene
- , John Berridge
- , Nicola Best
- , Debby Bogaert
- , Laura Booth
- , Pieter Bothma
- , Benjamin Brennan
- , Robin Brittain-Long
- , Katie Bullock
- , Naomi Bulteel
- , Tom Burden
- , Andrew Burtenshaw
- , Nicola Carlucci
- , Gail Carson
- , Vikki Caruth
- , Emily Cass
- , Benjamin W. A. Catterall
- , David Chadwick
- , Duncan Chambler
- , Meera Chand
- , Kanta Chechi
- , Nigel Chee
- , Jenny Child
- , Srikanth Chukkambotla
- , Richard Clark
- , Tom Clark
- , Jordan J. Clark
- , Emily A. Clarke
- , Sara Clohisey
- , Sarah Cole
- , Paul Collini
- , Marie Connor
- , Graham S. Cooke
- , Louise Cooper
- , Catherine Cosgrove
- , Audrey Coutts
- , Helen Cox
- , Jason Cupitt
- , Maria-Teresa Cutino-Moguel
- , Ana da Silva Filipe
- , Jo Dalton
- , Paul Dark
- , Christopher Davis
- , Chris Dawson
- , Thushan de Silva
- , Samir Dervisevic
- , Oslem Dincarslan
- , Alejandra Doce Carracedo
- , Cara Donegan
- , Lorna Donelly
- , Phil Donnison
- , Chloe Donohue
- , Gonçalo dos Santos Correia
- , Sam Douthwaite
- , Thomas M. Drake
- , Andrew Drummond
- , Marc-Emmanuel Dumas
- , Chris Dunn
- , Jake Dunning
- , Ingrid DuRand
- , Ahilanadan Dushianthan
- , Tristan Dyer
- , Philip Dyer
- , Angela Elliott
- , Cariad Evans
- , Anthony Evans
- , Chi Eziefula
- , Cameron J. Fairfield
- , Angie Fawkes
- , Chrisopher Fegan
- , Lorna Finch
- , Adam Finn
- , Lewis W. S. Fisher
- , Lisa Flaherty
- , Tom Fletcher
- , Terry Foster
- , Duncan Fullerton
- , Carrol Gamble
- , Isabel Garcia-Dorival
- , Atul Garg
- , Sanjeev Garg
- , Tammy Gilchrist
- , Michelle Girvan
- , Effrossyni Gkrania-Klotsas
- , Jo Godden
- , Arthur Goldsmith
- , Clive Graham
- , Tassos Grammatikopoulos
- , Christopher A. Green
- , Julian Griffin
- , Fiona Griffiths
- , Philip Gunning
- , Rishi K. Gupta
- , Katarzyna Hafezi
- , Sophie Halpin
- , Elaine Hardy
- , Ewen M. Harrison
- , Janet Harrison
- , Catherine Hartley
- , Stuart Hartshorn
- , Daniel Harvey
- , Peter Havalda
- , Daniel B. Hawcutt
- , Ross Hendry
- , Antonia Y. W. Ho
- , Maria Hobrok
- , Luke Hodgson
- , Karl Holden
- , Anthony Holmes
- , Peter W. Horby
- , Joanne Howard
- , Samreen Ijaz
- , Clare Jackson
- , Michael Jacobs
- , Susan Jain
- , Paul Jennings
- , Rebecca L. Jensen
- , Christopher B. Jones
- , Trevor R. Jones
- , Agilan Kaliappan
- , Vidya Kasipandian
- , Seán Keating
- , Stephen Kegg
- , Michael Kelsey
- , Jason Kendall
- , Caroline Kerrison
- , Ian Kerslake
- , Shadia Khandaker
- , Katharine King
- , Robyn T. Kiy
- , Stephen R. Knight
- , Susan Knight
- , Oliver Koch
- , Gouri Koduri
- , George Koshy
- , Chrysa Koukorava
- , Shondipon Laha
- , Eva Lahnsteiner
- , Steven Laird
- , Annette Lake
- , Suzannah Lant
- , Susan Larkin
- , Diane Latawiec
- , Andrew Law
- , James Lee
- , Gary Leeming
- , Daniella Lefteri
- , Tamas Leiner
- , Lauren Lett
- , Matthew Lewis
- , Sonia Liggi
- , Patrick Lillie
- , Wei Shen Lim
- , James Limb
- , Vanessa Linnett
- , Jeff Little
- , Lucia A. Livoti
- , Mark Lyttle
- , Louise MacGillivray
- , Alan Maclean
- , Michael MacMahon
- , Emily MacNaughton
- , Maria Mancini
- , Ravish Mankregod
- , Laura Marsh
- , Lynn Maslen
- , Hannah Massey
- , Huw Masson
- , Elijah Matovu
- , Nicole Maziere
- , Sarah McCafferty
- , Katherine McCullough
- , Sarah E. McDonald
- , Sarah McDonald
- , Laurence McEvoy
- , Ruth McEwen
- , John McLauchlan
- , Kenneth A. Mclean
- , Manjula Meda
- , Alexander J. Mentzer
- , Laura Merson
- , Soeren Metelmann
- , Alison M. Meynert
- , Nahida S. Miah
- , Joanna Middleton
- , Gary Mills
- , Jane Minton
- , Joyce Mitchell
- , Kavya Mohandas
- , James Moon
- , Elinoor Moore
- , Shona C. Moore
- , Patrick Morgan
- , Kirstie Morrice
- , Craig Morris
- , Katherine Mortimore
- , Samuel Moses
- , Mbiye Mpenge
- , Rohinton Mulla
- , Derek Murphy
- , Lee Murphy
- , Michael Murphy
- , Ellen G. Murphy
- , Thapas Nagarajan
- , Megan Nagel
- , Mark Nelson
- , Lisa Norman
- , Lillian Norris
- , Lucy Norris
- , Mahdad Noursadeghi
- , Michael Olanipekun
- , Wilna Oosthuyzen
- , Anthonia Osagie
- , Matthew K. O’Shea
- , Igor Otahal
- , Mark Pais
- , Massimo Palmarini
- , Carlo Palmieri
- , Selva Panchatsharam
- , Danai Papakonstantinou
- , Hassan Paraiso
- , Brij Patel
- , Natalie Pattison
- , William A. Paxton
- , Rebekah Penrice-Randal
- , Justin Pepperell
- , Mark Peters
- , Mandeep Phull
- , Jack Pilgrim
- , Stefania Pintus
- , Tim Planche
- , Daniel Plotkin
- , Georgios Pollakis
- , Frank Post
- , Nicholas Price
- , David Price
- , Tessa Prince
- , Rachel Prout
- , Nikolas Rae
- , Andrew Rambaut
- , Henrik Reschreiter
- , Tim Reynolds
- , Neil Richardson
- , P. Matthew Ridley
- , Mark Roberts
- , Stephanie Roberts
- , Devender Roberts
- , David L. Robertson
- , Alistair Rose
- , Guy Rousseau
- , Bobby Ruge
- , Clark D. Russell
- , Brendan Ryan
- , Debby Sales
- , Taranprit Saluja
- , Vanessa Sancho-Shimizu
- , Caroline Sands
- , Egle Saviciute
- , Matthias Schmid
- , Janet T. Scott
- , James Scott-Brown
- , Aarti Shah
- , Prad Shanmuga
- , Anil Sharma
- , Catherine A. Shaw
- , Victoria E. Shaw
- , Anna Shawcross
- , Rebecca K. Shears
- , Jagtur Singh Pooni
- , Jeremy Sizer
- , Benjamin Small
- , Richard Smith
- , Catherine Snelson
- , Tom Solomon
- , Rebecca G. Spencer
- , Nick Spittle
- , Shiranee Sriskandan
- , Nikki Staines
- , Tom Stambach
- , Richard Stewart
- , David Stuart
- , Krishanthi S. Subramaniam
- , Pradeep Subudhi
- , Olivia V. Swann
- , Tamas Szakmany
- , Agnieska Szemiel
- , Aislynn Taggart
- , Sarah Tait
- , Zoltan Takats
- , Panteleimon Takis
- , Jolanta Tanianis-Hughes
- , Kate Tatham
- , Richard S. Tedder
- , Jo Thomas
- , Jordan Thomas
- , Robert Thompson
- , Chris Thompson
- , Emma C. Thomson
- , Ascanio Tridente
- , Erwan Trochu
- , Darell Tupper-Carey
- , Lance C. W. Turtle
- , Mary Twagira
- , Nick Vallotton
- , Libby van Tonder
- , Rama Vancheeswaran
- , Rachel Vincent
- , Lisa Vincent-Smith
- , Shico Visuvanathan
- , Alan Vuylsteke
- , Sam Waddy
- , Rachel Wake
- , Andrew Walden
- , Ingeborg Welters
- , Murray Wham
- , Tony Whitehouse
- , Paul Whittaker
- , Ashley Whittington
- , Meme Wijesinghe
- , Eve Wilcock
- , Martin Williams
- , Lawrence Wilson
- , Stephen Winchester
- , Martin Wiselka
- , Adam Wolverson
- , Daniel G. Wootton
- , Andrew Workman
- , Nicola Wrobel
- , Bryan Yates
- , Peter Young
- , Maria Zambon
- & J. Eunice Zhang
Contributions
F.L. recruited participants, acquired clinical samples, analyzed and interpreted data and cowrote the manuscript, including all drafting and revisions. C.E. analyzed and interpreted data and cowrote this manuscript, including all drafting and revisions. S.F. and M.R. supported the analysis and interpretation of data as well as drafting and revisions. D.S., J.K.S., S.C.M., S.A., N.M., J.N., C.K., O.C.L., O.E., H.J.C.M., A. Shikotra, A. Singapuri, M.S., V.C.H., M.T., N.J.G., N.I.L. and C.C. contributed to acquisition of data underlying this study. L.H.-W., A.A.R.T., S.L.R.-J., L.S.H., O.M.K., D.G.W., T.I.d.S. and A. Ho made substantial contributions to conception/design and implementation of this work and/or acquisition of clinical samples for this work. They have supported drafting and revisions of the manuscript. E.M.H., J.K.Q. and A.B.D. made substantial contributions to the study design as well as data access, linkage and analysis. They have supported drafting and revisions of this work. J.D.C., L.-P.H., A. Horsley, B.R., K.P., M.M. and W.G. made substantial contributions to the conception and design of this work and have supported drafting and revisions of this work. J.K.B. obtained funding for ISARIC4C, is ISARIC4C consortium co-lead, has made substantial contributions to conception and design of this work and has supported drafting and revisions of this work. M.G.S. obtained funding for ISARIC4C, is ISARIC4C consortium co-lead, sponsor/protocol chief investigator, has made substantial contributions to conception and design of this work and has supported drafting and revisions of this work. R.A.E. and L.V.W. are co-leads of PHOSP-COVID, made substantial contributions to conception and design of this work, the acquisition and analysis of data, and have supported drafting and revisions of this work. C.B. is the chief investigator of PHOSP-COVID and has made substantial contributions to conception and design of this work. R.S.T. and L.T. made substantial contributions to the acquisition, analysis and interpretation of the data underlying this study and have contributed to drafting and revisions of this work. P.J.M.O. obtained funding for ISARIC4C, is ISARIC4C consortium co-lead, sponsor/protocol chief investigator and has made substantial contributions to conception and design of this work. R.S.T. and P.J.M.O. have also made key contributions to interpretation of data and have co-written this manuscript. All authors have read and approve the final version to be published. All authors agree to accountability for all aspects of this work. All investigators within ISARIC4C and the PHOSP-COVID consortia have made substantial contributions to the conception or design of this study and/or acquisition of data for this study. The full list of authors within these groups is available in Supplementary Information .
Corresponding authors
Correspondence to Ryan S. Thwaites or Peter J. M. Openshaw .
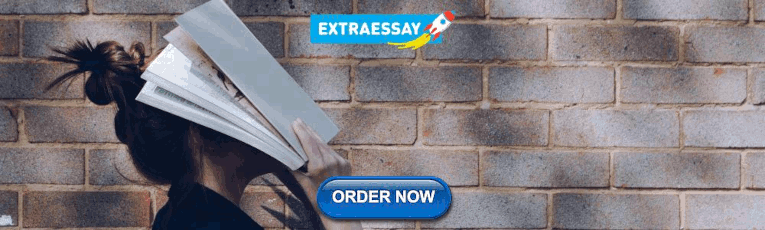
Ethics declarations
Competing interests.
F.L., C.E., D.S., J.K.S., S.C.M., C.D., C.K., N.M., L.N., E.M.H., A.B.D., J.K.Q., L.-P.H., K.P., L.S.H., O.M.K., S.F., T.I.d.S., D.G.W., R.S.T. and J.K.B. have no conflicts of interest. A.A.R.T. receives speaker fees and support to attend meetings from Janssen Pharmaceuticals. S.L.R.-J. is on the data safety monitoring board for Bexero trial in HIV+ adults in Kenya. J.D.C. is the deputy chief editor of the European Respiratory Journal and receives consulting fees from AstraZeneca, Boehringer Ingelheim, Chiesi, GSK, Insmed, Janssen, Novartis, Pfizer and Zambon. A. Horsley is deputy chair of NIHR Translational Research Collaboration (unpaid role). B.R. receives honoraria from Axcella therapeutics. R.A.E. is co-lead of PHOSP-COVID and receives fees from AstraZenaca/Evidera for consultancy on LC and from AstraZenaca for consultancy on digital health. R.A.E. has received speaker fees from Boehringer in June 2021 and has held a role as European Respiratory Society Assembly 01.02 Pulmonary Rehabilitation secretary. R.A.E. is on the American Thoracic Society Pulmonary Rehabilitation Assembly program committee. L.V.W. also receives funding from Orion pharma and GSK and holds contracts with Genentech and AstraZenaca. L.V.W. has received consulting fees from Galapagos and Boehringer, is on the data advisory board for Galapagos and is Associate Editor for the European Respiratory Journal . A. Ho is a member of NIHR Urgent Public Health Group (June 2020–March 2021). M.M. is an applicant on the PHOSP study funded by NIHR/DHSC. M.G.S. acts as an independent external and nonremunerated member of Pfizer’s External Data Monitoring Committee for their mRNA vaccine program(s), is Chair of Infectious Disease Scientific Advisory Board of Integrum Scientific LLC, and is director of MedEx Solutions Ltd. and majority owner of MedEx Solutions Ltd. and minority owner of Integrum Scientific LLC. M.G.S.’s institution has been in receipt of gifts from Chiesi Farmaceutici S.p.A. of Clinical Trial Investigational Medicinal Product without encumbrance and distribution of same to trial sites. M.G.S. is a nonrenumerated member of HMG UK New Emerging Respiratory Virus Threats Advisory Group and has previously been a nonrenumerated member of the Scientific Advisory Group for Emergencies (SAGE). C.B. has received consulting fees and/or grants from GSK, AstraZeneca, Genentech, Roche, Novartis, Sanofi, Regeneron, Chiesi, Mologic and 4DPharma. L.T. has received consulting fees from MHRA, AstraZeneca and Synairgen and speakers’ fees from Eisai Ltd., and support for conference attendance from AstraZeneca. L.T. has a patent pending with ZikaVac. P.J.M.O. reports grants from the EU Innovative Medicines Initiative 2 Joint Undertaking during the submitted work; grants from UK Medical Research Council, GSK, Wellcome Trust, EU Innovative Medicines Initiative, UK National Institute for Health Research and UK Research and Innovation–Department for Business, Energy and Industrial Strategy; and personal fees from Pfizer, Janssen and Seqirus, outside the submitted work.
Peer review
Peer review information.
Nature Immunology thanks Ziyad Al-Aly and the other, anonymous, reviewer(s) for their contribution to the peer review of this work. Ioana Staicu was the primary editor on this article and managed its editorial process and peer review in collaboration with the rest of the editorial team.
Additional information
Publisher’s note Springer Nature remains neutral with regard to jurisdictional claims in published maps and institutional affiliations.
Extended data
Extended data fig. 1 penalized logistic regression performance..
Graphs show classification error and Area under curve (AUC) from the 50 repeats tenfold nested cross-validation used to optimise and assess the performance of PLR testing associations with each LC outcome relative to Recovered (n = 233): Cardio_Resp (n = 398), Fatigue (n = 384), Anxiety/Depression (n = 202), GI (n = 132), ( e ) Cognitive (n = 6). The distributions of classification error and area under curve (AUC) from the nested cross-validation are shown. Box plot centre line represents the Median and boundaries of the box represent interquartile range (IQR), the whisker length represent 1.5xIQR.
Extended Data Fig. 2 Associations with long COVID symptoms in full study cohort.
( a ) Fibrinogen levels at 6 months were compared between pooled LC cases (n = 295) and Recovered (n = 233) and between the Cognitive group (n = 41) and Recovered (n = 233). Box plot centre line represent the Median and boundaries of the box represent interquartile range (IQR), the whisker length represents 1.5xIQR, any outliers beyond the whisker range are shown as individual dots. Median differences were compared using two-sided Wilcoxon signed-rank test *= p < 0·05, **= p < 0·01, ***= p < 0·001, ****= p < 0·0001. Unadjusted p-values are reported. b ) Distribution of time from COVID-19 hospitalisation at sample collection applying CDC and NICE definitions of LC (n = 719) ( c ) Upset plot of symptom groups. Horizontal coloured bars represent the number of patients in each symptom group: Cardiorespiratory (Cardio_Resp), Fatigue, Cognitive, Gastrointestinal (GI) and Anxiety/Depression (Anx_Dep). Vertical black bars represent the number of patients in each symptom combination group. To prevent patient identification, where less than 5 patients belong to a combination group, this has been represented as ‘<5’. The Recovered group (n = 250) were used as controls. Forest plots show Olink protein concentrations (NPX) associated with ( d ) Cardio_Resp (n = 398), ( e ) Fatigue (n = 342), ( f ) Anx_Dep (n = 219), ( g ) GI (n = 134), and ( h ) Cognitive (n = 65). Error bars represent the median accuracy of the model.
Extended Data Fig. 3 Validation of olink measurements using conventional assays in plasma.
Olink measured protein (NPX) were compared to chemiluminescence assays (ECL or ELISA, log2[pg/mL]) to validate our findings, where contemporaneously collected plasma samples were available (n = 58). Results from key mediators associated with LC groups were validated: CSF3, IL1R2, IL2, IL3RA, TNFa, TFF2. R = spearman rank correlation coefficient and shaded areas indicated the 95% confidence interval. Samples that fell below the lower limit of detection for a given assay were excluded and the ‘n’ value on each panel indicates the number of samples above this limit.
Extended Data Fig. 4 Univariate analysis of proteins associated with each symptom.
Olink measured plasma protein levels (NPX) compared between LC groups (Cardio_Resp, n = 398, Fatigue n = 384, Anxiety/Depression, n = 202, GI, n = 132 and Cognitive, n = 60) and Recovered (n = 233). Proteins identified by PLR were compared between groups. Median differences were compared using two-sided Wilcoxon signed-rank test. * = p < 0·05, ** = p < 0·01, *** = p < 0·001, ****= p < 0·0001 after FDR adjustment. Box plot centre line represent the Median and boundaries of the box represent interquartile range (IQR), the whisker length represents 1.5xIQR, any outliers beyond the whisker range are shown as individual dots.
Extended Data Fig. 5 Unadjusted Penalised Logistic Regression.
Olink measured proteins (NPX) and their association with Cardio_Resp (n = 398), Fatigue (n = 342), Anx_Dep (n = 219), GI (n = 134), and Cognitive (n = 65). Forest plots show odds of each LC outcome vs Recovered (n = 233), using PLR without adjusting for clinical co-variates. Error bars represent the median accuracy of the model.
Extended Data Fig. 6 Partial Least Squares analysis.
Olink measured proteins (NPX) and their association with Cardio_Resp (n = 398), Fatigue (n = 342), Anx_Dep (n = 219), GI (n = 134), and Cognitive (n = 65) groups. Forest plots show odds of LC outcome vs Recovered (n = 233), using PLS analysis. Error bars represent the standard error of the coefficient estimate.
Extended Data Fig. 7 Network analysis centrality.
Each graph shows the centrality score for each Olink measured protein (NPX) found to have significant associations with other proteins that were elevated in the Cardio_Resp (n = 398), Fatigue (n = 342), Anx_Dep (n = 219), GI (n = 134), and Cognitive (n = 65) groups relative to Recovered (n = 233).
Extended Data Fig. 8 Inflammation in men and women with long COVID.
Olink measured plasma protein levels (NPX) between men and women with symptoms, divided by age (<50 or >=50years): (a) shows IL1R2 and MATN2 in the Anxiety/Depression group (<50 n = 55, >=50 n = 133), (b) shows CTSO and NFASC in the Cognitive group (<50 n = 11, >=50 n = 50). Median values were compared between men and women using two-sided Wilcoxon signed-rank test. Box plot centre line represent the Median and boundaries represent interquartile range (IQR), the whisker length represents 1.5xIQR.
Extended Data Fig. 9 Inflammation in the upper respiratory tract.
Nasal cytokines measured by immunoassay in the CardioResp Group (n = 29) and Recovered (n = 31): ( a ) shows IL1a, IL1b, IL-6, APO-2, TGFa, TFF2. Median differences were compared using two-sided Wilcoxon signed-rank test. Box plot centre line represents the Median and boundaries of the box represent interquartile range (IQR), the whisker length represent 1.5xIQR. ( b ) Shows cytokines measured by immunoassay in paired plasma and nasal (n = 70). Correlations between IL1a, IL1b, IL-6, APO-2, TGFa and TFF2 in nasal and plasma samples were compared using Spearman’s rank correlation coefficient ( R ). Shaded areas indicated the 95% confidence interval of R.
Extended Data Fig. 10 Graphical abstract.
Summary of interpretation of key findings from Olink measured proteins and their association with CardioResp (n = 398), Fatigue (n = 342), Anx/Dep (n = 219), GI (n = 134), and Cognitive (n = 65) groups relative to Recovered (n = 233).
Supplementary information
Supplementary information.
Supplementary Methods, Statistics and reproducibility statement, Supplementary Results, Supplementary Tables 1–7, Extended data figure legends, Appendix 1 (Supplementary Table 8), Appendix 2 (PHOSP-COVID author list) and Appendix 3 (ISARIC4C author list).
Reporting Summary
Rights and permissions.
Open Access This article is licensed under a Creative Commons Attribution 4.0 International License, which permits use, sharing, adaptation, distribution and reproduction in any medium or format, as long as you give appropriate credit to the original author(s) and the source, provide a link to the Creative Commons licence, and indicate if changes were made. The images or other third party material in this article are included in the article’s Creative Commons licence, unless indicated otherwise in a credit line to the material. If material is not included in the article’s Creative Commons licence and your intended use is not permitted by statutory regulation or exceeds the permitted use, you will need to obtain permission directly from the copyright holder. To view a copy of this licence, visit http://creativecommons.org/licenses/by/4.0/ .
Reprints and permissions
About this article
Cite this article.
Liew, F., Efstathiou, C., Fontanella, S. et al. Large-scale phenotyping of patients with long COVID post-hospitalization reveals mechanistic subtypes of disease. Nat Immunol 25 , 607–621 (2024). https://doi.org/10.1038/s41590-024-01778-0
Download citation
Received : 11 August 2023
Accepted : 06 February 2024
Published : 08 April 2024
Issue Date : April 2024
DOI : https://doi.org/10.1038/s41590-024-01778-0
Share this article
Anyone you share the following link with will be able to read this content:
Sorry, a shareable link is not currently available for this article.
Provided by the Springer Nature SharedIt content-sharing initiative
This article is cited by
Immune dysregulation in long covid.
- Laura Ceglarek
- Onur Boyman
Nature Immunology (2024)
Quick links
- Explore articles by subject
- Guide to authors
- Editorial policies
Sign up for the Nature Briefing newsletter — what matters in science, free to your inbox daily.

REVIEW article
This article is part of the research topic.
Advanced Biomaterials for Hard Tissue Repair and Regeneration
Emerging roles of hydrogel in promoting periodontal tissue regeneration and repairing bone defect Provisionally Accepted
- 1 Department of Prosthodontics and Implant Dentistry, First Affiliated Hospital of Xinjiang Medical University, China
- 2 Affiliated Stomatological Hospital of Xinjiang Medical University, China
- 3 Stomatology Research Institute of Xinjiang Uygur Autonomous Region, China
The final, formatted version of the article will be published soon.
Periodontal disease is the most common type of oral disease. Periodontal bone defect is the clinical outcome of advanced periodontal disease, which seriously affects the quality of life of patients. Promoting periodontal tissue regeneration and repairing periodontal bone defects is the ultimate treatment goal for periodontal disease, but the means and methods are very limited. Hydrogels are a class of highly hydrophilic polymer networks, and their good biocompatibility has made them a popular research material in the field of oral medicine in recent years. This paper reviews the current mainstream types and characteristics of hydrogels, and summarizes the relevant basic research on hydrogels in promoting periodontal tissue regeneration and bone defect repair in recent years. The possible mechanisms of action and efficacy evaluation are discussed in depth, and the application prospects are also discussed.
Keywords: Hydrogel, Periodontal tissue regeneration, Bone Tissue Engineering (bone-TE), Repair (E), Bone defect
Received: 01 Feb 2024; Accepted: 08 Apr 2024.
Copyright: © 2024 Guo, Dong and Wang. This is an open-access article distributed under the terms of the Creative Commons Attribution License (CC BY) . The use, distribution or reproduction in other forums is permitted, provided the original author(s) or licensor are credited and that the original publication in this journal is cited, in accordance with accepted academic practice. No use, distribution or reproduction is permitted which does not comply with these terms.
* Correspondence: Dr. Xing Wang, First Affiliated Hospital of Xinjiang Medical University, Department of Prosthodontics and Implant Dentistry, Urumqi, China
People also looked at
Team publishes paper on tissue deformation tracking
Cristian Linte , associate professor; Richard Simon , research scientist; Zixin Yang ; and Kelly Merrell , from the Chester F. Carlson Center for Imaging Science and Department of Biomedical Engineering, published “Boundary Constraint-free Biomechanical Model-Based Surface Matching for Intraoperative Liver Deformation Correction,” with support from the National Science Foundation. The team developed a novel 3D-3D non-rigid registration method that outperforms existing techniques, ensuring accurate tissue deformation tracking without requiring zero-boundary conditions or specific force locations.
Recommended News
April 12, 2024
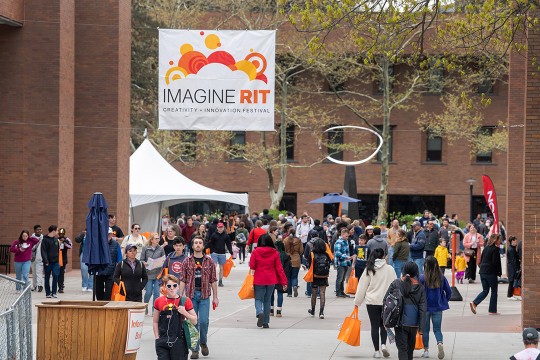
Imagine RIT: Creativity and Innovation Festival set for April 27
Detecting deepfakes, a device to help grocery stores like Wegmans inspect strawberries and reduce waste, electric wheelchairs that run on brainwaves, and even the economic impact of Taylor Swift are just a few of the nearly 400 exhibits at this year’s Imagine RIT: Creativity and Innovation Festival.
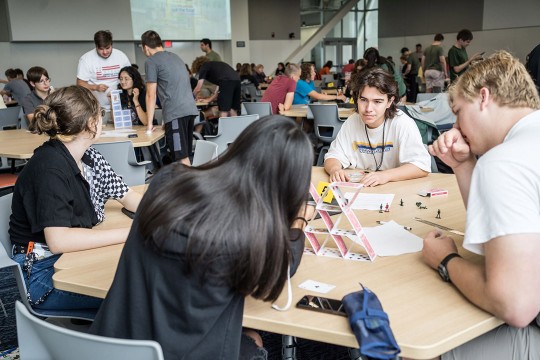
RIT offers Summer Institute for Teaching and Learning and AI Symposium May 14-15
Collaborative, self-directed, and socially conscious are just a few of the characteristics that enable 21st-century students to thrive in today’s world. At RIT’s Summer Institute, educators are coming together to discuss best practices for teaching the next generation.
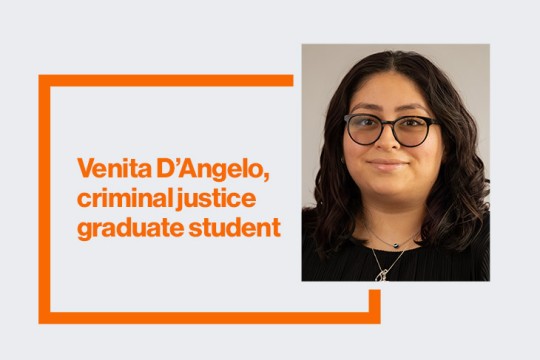
RIT graduate student gains experience through Center for Public Safety Initiatives
Venita D’Angelo, a graduate student pursuing a master’s degree in criminal justice, is working to reduce crime through program evaluation, data analytics, and project management services for area law enforcement, community nonprofits, and other criminal justice professionals.
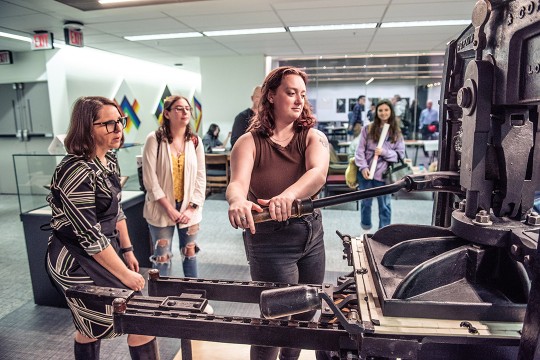
Cary Collection opening Thursday was grand
What do Nicolas Copernicus, William Morris, and Joe Kubert have in common? Works by the astronomer, designer, and comics artist—legends in their respective fields—are represented in the Cary Graphic Arts Collection and are now accessible to everyone in the remodeled Wallace Library.

An official website of the United States government
The .gov means it’s official. Federal government websites often end in .gov or .mil. Before sharing sensitive information, make sure you’re on a federal government site.
The site is secure. The https:// ensures that you are connecting to the official website and that any information you provide is encrypted and transmitted securely.
- Publications
- Account settings
Preview improvements coming to the PMC website in October 2024. Learn More or Try it out now .
- Advanced Search
- Journal List
- v.29; 2020 Apr
Characterization data of pulp fibres performance in tissue papers applications
Flávia p. morais.
a FibEnTech - Fiber Materials and Environmental Technologies Research Unit, University of Beira Interior, Covilhã, Portugal
Raquel A.C. Bértolo
b RAIZ - Forest and Paper Research Institute, Aveiro, Portugal
Joana M.R. Curto
c CIEPQPF - Chemical Process Engineering and Forest Products Research Centre, Department of Chemical Engineering, University of Coimbra, Coimbra, Portugal
Maria E.C.C. Amaral
Ana m.m.s. carta, dmitry v. evtyugin.
d CICECO/Department of Chemistry, University of Aveiro, Aveiro, Portugal
Associated Data
The data presented in this article are related to the original research paper entitled “Comparative characterization of eucalyptus fibres and softwood fibres for tissue papers applications” available in Materials Letter: X Journal [1]. In this article, six eucalyptus hardwood pulps and six softwood pulps were characterized in terms of morphological, chemical and water-related (by drainability and water retention index) properties. In addition, using these pulps, unpressed laboratory isotropic handsheets were produced with a basis weight of approximately 20 g/m 2 , similarly to tissue papers. The key properties of tissue papers, namely structural properties, tensile index, absorption, and handfeel softness were analysed in these handsheets.
Specifications Table
Here we report experimental characterization data on six eucalyptus hardwood and six softwood pulps and un-pressed laboratory isotropic handsheets with a basis weight of approximately 20 g/m 2 [ 1 ]. Analysis of fibres morphology, chemical properties, drainability (Schöpper-Riegler degree - °SR), water retention value (WRV), structural, tensile, absorption and handfeel (HF) softness tissue properties are shown ( Table 1 ). Some correlations found about these properties also are shown, namely tensile index versus curl and kinked fibres ( Fig. 1 ) and tensile index versus softness ( Fig. 2 ).
Fibers morphology, chemical composition, and tissue paper properties characterization for hardwood and softwood pulps.

Correlation of tensile index and curl and kinked fibres for hardwood and softwood pulps.

Correlation of tensile index and softness for hardwood and softwood pulps.
2. Experimental design, materials, and methods
2.1. pulp samples.
Twelve industrial kraft pulps (six eucalyptus hardwood and six softwood pulps), with different bleaching sequences, were analysed. For all samples, the dry matter content was determined by placing the samples on an infrared scale at 105 °C for 30 minutes, following an adaptation of ISO 638 standard.
2.2. Fibres morphological properties and pulps composition
The pulps morphological analysis was performed using a system based on image analysis, namely MorFi® (TECHPAP, Grenoble, France) equipment. The equipment integrates a digital camera and image analysis software for the automatic measurement of suspended fibres. Parameters such as length, width, coarseness, curl, kinks and fines were analysed. All assays were done in triplicate.
2.3. Chemical characterization
The pulp's viscosity, the pentosan content and the carboxyl group content was determined according to SCAN-CM 15:88, TAPPI T 223 cm-10, TAPPI T 237 om-97 standard, respectively.
2.4. Pulps suspension characterization
The pulps drainability was evaluated considering the measurement of the Schopper-Riegler degree according to ISO 5267/1 standard. For each sample, triplicate assays were performed.
The Water Retention Value (WRV) was determined according to the method described by Jayme [ 2 ]. This method is based on centrifugation at 7000 revolutions per minute, about 2 g of wet pulp for 10 minutes. Thereafter, the already centrifuged pulp was removed and weighed exactly to the tenth of a milligram. Finally, centrifuged pulp samples were dried in the oven (105 ± 2 °C) for further determination of the sample dry weight. For each sample, triplicate assays were performed.
2.5. Laboratory isotropic handsheets preparation and testing
The handsheets of each pulp were produced according to an adaptation of ISO 5269-1 standard. Handsheets with a basis weight of approximately 20 g/m 2 and unpressed were produced to approximate more tissue paper, using a laboratory handsheet former according to the respective standard. Finally, the handsheets were removed from the former web with a blotting paper and placed in a conditioned room (temperature of 23.0 ± 1.0 °C and relative humidity of 50.0 ± 2.0%). For each assay, 10 replicates were carried out for each sample.
The handsheets produced were tested for various tissue paper properties like, thickness and bulk (ISO 12625-3), basis weight (ISO 12625-6), tensile index (ISO 12625-4), water absorption capacity (ISO 12625-8), and softness using a TSA - Tissue Softness Analyzer (Emtec) equipment. The handsheets porosity was also determined by the Henriksson et al. [ 3 ] equation:
where ρ handsheets corresponds to the handsheet's apparent density and ρ cellulose corresponds to the cellulose density (1.5 g/cm 3 ).
Acknowledgments
This research was supported by Project InPaCTus – Innovative Products and Technologies from eucalyptus, Project Nº 21 874 funded by Portugal 2020 through European Regional Development Fund (ERDF) in the frame of COMPETE 2020 nº 246/AXIS II/2017.
Appendix A Supplementary data to this article can be found online at https://doi.org/10.1016/j.dib.2020.105253 .
Conflict of Interest
The authors declare that they have no known competing financial interests or personal relationships that could have appeared to influence the work reported in this paper.
Appendix A. Supplementary data
The following is the Supplementary data to this article:
Emerging Treatments for Childhood Interstitial Lung Disease
- Leading Article
- Open access
- Published: 10 November 2023
- Volume 26 , pages 19–30, ( 2024 )
Cite this article
You have full access to this open access article
- Nicol Bernardinello 1 ,
- Matthias Griese 2 ,
- Raphaël Borie 3 &
- Paolo Spagnolo 1
1540 Accesses
Explore all metrics
Childhood interstitial lung disease (chILD) is a large and heterogeneous group of disorders characterized by diffuse lung parenchymal markings on chest imaging and clinical signs such as dyspnea and hypoxemia from functional impairment. While some children already present in the neonatal period with interstitial lung disease (ILD), others develop ILD during their childhood and adolescence. A timely and accurate diagnosis is essential to gauge treatment and improve prognosis. Supportive care can reduce symptoms and positively influence patients' quality of life; however, there is no cure for many of the chILDs. Current therapeutic options include anti-inflammatory or immunosuppressive drugs. Due to the rarity of the conditions and paucity of research in this field, most treatments are empirical and based on case series, and less than a handful of small, randomized trials have been conducted thus far. A trial on hydroxychloroquine yielded good safety but a much smaller effect size than anticipated. A trial in fibrotic disease with the multitargeted tyrosine kinase inhibitor nintedanib showed similar pharmacokinetics and safety as in adults. The unmet need for the treatment of chILDs remains high. This article summarizes current treatments and explores potential therapeutic options for patients suffering from chILD.
Similar content being viewed by others
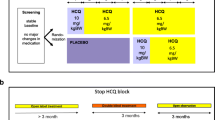
Prospective evaluation of hydroxychloroquine in pediatric interstitial lung diseases: Study protocol for an investigator-initiated, randomized controlled, parallel-group clinical trial
Matthias Griese, Meike Köhler, … Elias Seidl
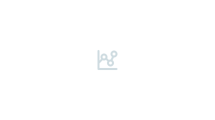
Rare Lung Diseases: Interstitial Lung Diseases and Lung Manifestations of Rheumatological Diseases
Mahesh babu Ramamurthy, Daniel Y.T. Goh & Michael Teik Chung Lim
Current and Emerging Treatment Options in Interstitial Lung Disease
Avoid common mistakes on your manuscript.
1 Introduction
The term childhood interstitial lung disease (chILD) was coined to collect a large and heterogeneous group of rare and ultra-rare entities manifesting during childhood. Despite being uncommon, the burden of chILD is high for both caregivers and the health system [ 1 , 2 ]. Indeed, chILD has a high morbidity and mortality rate, and a global prevalence of 1.6–46 per million [ 3 , 4 , 5 , 6 ]. Interstitial lung disease (ILD) in children is approximately ten times rarer and, at the same time, 100 times less studied and published than adult ILD [ 7 ]. The diagnosis of chILD should be suspected if at least three of the following four elements are present for more than 4 weeks in the absence of a respiratory tract infection: (1) symptoms like exercise intolerance, tachypnea, (dry) cough; (2) respiratory signs including dyspnea, crackles on lung auscultation, failure to thrive; (3) respiratory insufficiency with hypoxia/low oxygen saturation; and (4) diffuse parenchymal lung abnormalities on chest computed tomography (CT) scan or chest X-ray [ 8 ].
When chILD is suspected, a detailed anamnesis and family history (with emphasis on siblings/relatives with a history of ILD or early death from lung disease), clinical examination searching for potential rheumatological, immunological, or dermatological manifestations, and a chest X-ray should be performed, before referring the patient to an expert center. Additional clinical signs may include wall deformity or pectus excavatum (for example, in patients with surfactant disorders), or digital clubbing.
As with adult ILD, the diagnostic algorithm includes non-invasive (i.e., pulmonary function tests, assessment of ventilation and oxygenation, and chest imaging) and invasive procedures (i.e., bronchoscopy, and lung biopsy). Moreover, genetic testing has been implemented in most expert centers, thus improving diagnostic accuracy and reducing the need for invasive diagnostic modalities. Despite considerable progress in recent years, disease pathogenesis remains unknown in many of the chILDs, making the development of efficacious treatments challenging [ 9 , 10 ]. Moreover, the rarity of each condition and the fact that patients are often living in geographically dispersed areas represent additional important hurdles when planning clinical trials among many other obstacles [ 11 ]. In this regard, the importance of collaboration among expert centers with the aim of establishing patient registries and databases cannot be overemphasized [ 12 ]. In this review, we explore the landscape of pharmacological treatment of chILD.
2 ChILD Classification
Although the term chILD has the advantage of allowing easy communication and is popular, it has several disadvantages, including lumping together many conditions with completely different presentations, treatments, and outcomes, even including conditions involving the lung interstitium only indirectly or very mildly, like in patients with persistent tachypnea of infancy [ 13 ]. Thus, the need for a simple classification system emerged over the years. In 2004, a task force conducted by the European Respiratory Society, which included respiratory physicians and basic scientists, reviewed 185 cases of chILD and classified them with a system similar to that used for adult disease [ 14 ]. A few years later, a new classification scheme based on lung histology was proposed for ILD in children < 2 years of age [ 15 , 16 ]. This classification system was later extended to all pediatric age groups [ 17 ]. Recently, an etiological classification system combining pediatric and adult lung ILD in a single system was proposed [ 18 ]. The system differentiates four main categories, i.e., lung‐only (native parenchymal) disorders, systemic disease‐related disorders, exposure-related disorders, and vascular disorders. Of particular importance are those conditions closely linked to lung development and thus representing the majority of “typical” chILD, such as those in children not surviving into adulthood, those infrequently diagnosed at adult age, and those that transition into adulthood, as is now being seen more and more [ 19 ]. They include “Diffuse developmental disorders (A1),” usually resulting in death within the neonatal period; “Growth abnormalities with deficient alveolarization (A2),” such as lung hypoplasia, chronic lung disease of prematurity (bronchopulmonary dysplasia [BPD]), and others with a somewhat better prognosis; and “Infant conditions of undefined etiology (A3),” comprising the overall most frequent diagnoses in these children, i.e., neuroendocrine cell hyperplasia of infancy (NEHI) (or more correctly labeled as persistent tachypnoea of infancy [PTI]) [ 13 , 20 , 21 ]. Lastly, “ILD related to the alveolar surfactant region (A4)” includes surfactant dysfunction disorders [ 22 ] and pulmonary alveolar proteinosis (PAP) [ 23 ]. Patients with the latter diagnosis now often reach adulthood or are diagnosed at adult age [ 24 , 25 , 26 ]. Many other conditions, in particular “ILD related to systemic disease processes (B1),” with examples like sarcoidosis [ 27 ] and connective tissue diseases [ 28 ], are ILDs with increasing frequency in adulthood.
Recently, the Children's Interstitial and Diffuse Lung Disease Research Network (chILDRN) published data regarding a prospective registry including 683 individuals enrolled from different centers in the United States. NEHI was the most frequent diagnosis (23%), the second being ILD associated with connective tissue or immune‐mediated disorders (16.5%). Notably, 11% of cases of chILD remained “unclassified” (Table 1 ) [ 29 ].
3 Genetic Background
Despite intrinsic peculiarities, chILDs often share similar clinical and radiological manifestations and may be difficult to differentiate from one another. Genetic testing can identify disease-causing mutations, thus reducing the need for invasive diagnostic procedures (Table 2 ) [ 30 ]. The number of genetic etiologies identified as causes of ILD in children continues to grow and includes genes involved in surfactant production ( SFTPB, SFTPC , ABCA3 , and NKX2-1 ) [ 31 , 32 , 33 , 34 , 35 ] and catabolism ( CSF2RA and CSF2RB ) [ 36 , 37 ], immune regulation ( COPA ) [ 38 , 39 ], and lung development ( FLNA and TBX4 , among others) [ 40 , 41 ]. Some chILDs are associated with high mortality, whereas others have a favorable outcome. For instance, surfactant protein B (SP-B) deficiency has the worst prognosis, whereas variants within SFTPC may lead to a range of phenotypes and prognoses (Fig. 1 ) [ 42 ]. On the contrary, most but not all children with PTI/NEHI tend to experience a favorable prognosis; therefore, a limited follow-up to 10 or 15 years of age needs to be considered [ 43 ].
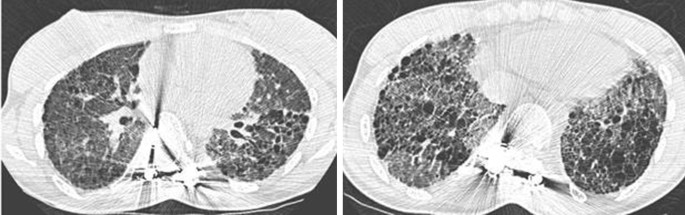
Seventeen-year-old girl with SP-C deficiency and interstitial lung disease. CT scans show extensive ground glass opacity and traction bronchiectasis throughout both lungs. CT computed tomography, SP-C surfactant protein C
4 Current Therapeutic Options for ChILD
Corticosteroids, hydroxychloroquine, and azithromycin are the most common pharmacological treatments for patients with chILD [ 44 ]. Less frequently used medications include azathioprine, cyclophosphamide, and colchicine [ 45 ]. Acute exacerbations in chILD were treated with β-lactam antibiotics (54%), systemic glucocorticosteroids (25%), inhaled bronchodilators (24%), or macrolides (19%) [ 46 ].
Intravenous pulse methylprednisolone (10–30 mg/kg) is used in critical patients or those needing ventilation [ 47 ]. Chronic treatments with oral glucocorticosteroids in children are done rarely, and the frequency and (potential) severity of side effects of corticosteroids must always be considered [ 48 ]. Consequently, close clinical monitoring is mandatory, including bone density, periodic complete blood count, and growth measurements [ 49 ].
Hydroxychloroquine is an antimalarial drug used in several autoimmune diseases, including arthritis [ 50 ]; historically, it has been used also in some chILDs, although its role in this disease remains controversial. Between 1984 and 2013, 85 case reports and small case series reported on children with different forms of ILD who were treated with chloroquine or hydroxychloroquine. In 35 cases, the effect was beneficial, while in the remaining, the results were unsatisfactory or inconclusive [ 51 ]. Hydroxychloroquine is generally safe, but its chronic use may be associated with visual loss, making regular visual assessment mandatory [ 52 ]. The effect of hydroxychloroquine in patients with chILD has been recently evaluated in a phase 2a, randomized, double-blind, placebo-controlled, multinational study [ 53 , 54 ]. The primary endpoint of presence or absence of response to treatment as assessed by oxygenation (calculated from a change in transcutaneous O 2 saturation of ≥ 5%, respiratory rate ≥ 20%, or level of respiratory support) did not differ between hydroxychloroquine and placebo. Acknowledging major limitations such as the small study population ( n = 26), the heterogeneity of included patients, the treatment duration (12 weeks followed by an open observation period of 12 weeks), and the lack of lung function data below the age of 6 years, the authors warned that prescription of hydroxychloroquine in daily practice needs to be reassessed. It is very likely that the beneficial effect of hydroxychloroquine is mutation specific, particularly in ABCA3 deficiency [ 55 ].
Azithromycin is an anti-inflammatory and immunomodulatory antibiotic that is used in a range of respiratory diseases; however, the evidence favoring its utility in chILD is limited to less than a handful of case reports [ 56 ]. Although widely used, no clinical trial of azithromycin has been conducted in chILD, and its role remains marginal and empirical; the potential risk of microbial resistance to azithromycin should also be considered [ 57 ]. Although prospective data are needed, several case reports have reported improvements of adult ILD associated with ABCA3 deficiency following azithromycin treatment, suggesting a possible benefit to be evaluated in selected chILDs [ 24 , 25 , 26 , 27 , 28 , 29 , 30 , 31 , 32 , 33 , 34 , 35 , 36 , 37 , 38 , 39 , 40 , 41 , 42 , 43 , 44 , 45 , 46 , 47 , 48 , 49 , 50 , 51 , 52 , 53 , 54 , 55 , 56 , 57 ,, 58 ].
Whole lung lavage (WLL) is the standard treatment in PAP, but this procedure is available only in specialized centers. Indeed, special techniques are necessary in infants, due to the small airway dimensions [ 59 , 60 , 61 ]. In addition, although safe and efficacious, WLL is invasive and time-consuming [ 62 ].
Specific therapies with a mechanistically plausible role exist, but they are applicable only in a minority of conditions (biologics [i.e., rituximab], immunomodulatory therapies in connective tissue disorders, or stem cell transplant in alveolar proteinosis) [ 63 , 64 ].
5 Non-pharmacological Treatments
Supportive care is similarly important for children with ILD. As with adult disease, chILD patients with gas exchange impairment may benefit from supplemental oxygen, whereas children with severe respiratory failure may benefit from invasive or non-invasive ventilation [ 65 ]. Patients with chILD may display poor somatic growth, thus needing specific nutritional support. Lessons learned from BPD and cystic fibrosis (CF) suggest that growth should be closely monitored also in patients with chILD.
Similar to adult ILD patients, preventing further damage to the lung is critically important. Pneumonia and other infections impart an important morbidity and mortality burden on children with ILD. Therefore, vaccination (pneumococcal and annual influenza), avoidance of harmful environmental exposures (such as second-hand smoke), and appropriate personal hygiene (both for children and caregivers) are strongly recommended [ 16 ].
Gastroesophageal reflux disease (GERD), a common comorbidity in adult ILD patients, has been investigated also in chILD. In a study by Dziekiewicz and colleagues, the prevalence of GERD among children with ILD ( n = 18) aged 0.2–11.6 years was 50% [ 66 ].
Lung transplantation is rarely indicated but has been successfully performed in cases of SP-B and SP-C deficiency, in patients carrying variants within ABCA3 and NKX2-1 , and in children with chronic pneumonitis of infancy. In comparison with adolescents, children are more often transplanted for ILD and precapillary pulmonary hypertension than for respiratory diseases, but with a similar in-hospital mortality [ 67 ]. A single-lobe lung transplantation has been successfully performed from a living donor to a patient with ABCA3 disorder [ 68 ].
6 Treatment of Specific Conditions
To date, there is no therapy specifically approved for chILD. However, in 2015, the chILD-EU collaboration created standard operating procedures and protocols for a staged investigation of chILD. In addition, participating centers across Europe developed a Delphi consensus process with the aim of harmonizing treatment protocols such as the use of intravenous and oral corticosteroids, and add-on therapies such as hydroxychloroquine and azithromycin [ 17 ]. Thus, the development of efficacious and well-tolerated drugs is a particularly urgent need [ 69 ]. Because of the rarity of these diseases, many barriers exist to drug development for chILD, including the low economic benefits for the pharma industry and limited funding for researchers. Yet, the burden of chILD on the healthcare system is very high [ 1 ]. At present, the management of patients with chILD largely relies on case series investigating the effect of anti-inflammatory and immunomodulatory drugs to prevent the development of lung fibrosis. In this regard, most of the drugs used for chILD derive from the treatment of adult disease [ 70 ].
6.1 Fibrosing ILD
Two drugs with pleiotropic antifibrotic effects (pirfenidone and nintedanib) can reduce the rate of functional decline—as assessed by forced vital capacity (FVC)—and disease progression in adult patients with idiopathic pulmonary fibrosis (IPF) [ 71 , 72 ] and ILD that progress despite appropriate treatment (nintedanib) [ 73 ]. The similarities between childhood and adult diseases provided the rationale for assessing the efficacy of antifibrotic drugs approved for adult diseases also in chILD. In a recent phase 2, double-blind, randomized, placebo-controlled trial (NCT04093024—InPedILD trial) [ 74 ], 39 patients aged 6–17 years with fibrosing ILD on chest CT and clinically significant disease were randomized 2:1 to receive nintedanib ( n = 26) or placebo ( n = 13) for 24 weeks. Co-primary endpoints were the area under the plasma concentration–time curve at steady state at weeks 2 and 26 and the proportion of patients with treatment-emergent adverse events at week 24. Two patients (7.7%) discontinued nintedanib because of adverse events. As with adult patients, diarrhea was the most frequent adverse event associated with nintedanib, being reported in 38.5% of cases (compared to 15.4% in the placebo group). Mean change in FVC % predicted at week 24 was 0.3% in the nintedanib group versus −0.9% in the placebo group. A stabilization of peripheral oxygen saturation (SpO 2 ) at rest over 24 weeks in the nintedanib group was also observed; both results were not statistically significant, although the study was not powered to assess efficacy. Overall, in children and adolescent with fibrosing ILD, a weight-based regimen resulted in exposure to nintedanib similar to adult patients, with an acceptable safety and tolerability profile. An open-label extension to assess long-term safety and tolerability of nintedanib in children and adolescents with ILD is currently recruiting (NCT05285982) [ 75 ].
6.2 Telomere-Related Diseases
Similar to adult disease, short telomeres and variations in telomere-related genes can manifest as ILD also in children [ 76 , 77 ]. A phase 1/2 study has shown that danazol, a synthetic sex hormone with androgenic properties, leads to telomere elongation in patients with telomere diseases (NCT01441037) [ 78 ]. A multicenter, phase 2, double-blind, placebo-control trial that is currently ongoing will assess the safety and efficacy of danazol (in combination with standard of care) in adults and pediatric patients with pulmonary fibrosis associated with short telomeres. With regard to the pediatric population (age < 16 years), enrollment in the trial is limited to patients with a diagnosis of dyskeratosis congenita who will receive danazol 2 mg/kg/day, while adult patients will receive a dosage of 4 mg/kg/day (TELOSCOPE—NCT04638517) [ 79 ]. The primary endpoint is the annual change in absolute telomere length from baseline. The efficacy of danazol is also investigated in the French ANDROTELO study (NCT03710356). The results should be available soon, but without the chILD population.
6.3 Disorders of Surfactant Dysfunction
Ivacaftor and genistein, two drugs approved for CF, have been evaluated as potential treatments in patients carrying ABCA3 variants, the rationale being that the ABCA3 gene has some degree of homology with CFTR , with the cystic fibrosis transmembrane conductance regulator (CFTR) also being an ABC transporter (Fig. 2 ). In CF, both ivacaftor and genistein increase CFTR channel opening, which translates to several beneficial effects, including lung function, surfactant function, weight gain, and fertility. Disorders related to ABCA3 dysfunction lead to respiratory distress syndrome, early death, and chronic ILD in children and adults [ 24 ]. Kinting and colleagues have shown that disease-causing misfolding ABCA3 variants can be rescued in vitro by the bithiazole correctors C13 and C17 as well as by the chemical chaperone trimethylamine N -oxide and low temperature [ 80 ]. Moreover, in A549 cells expressing ABCA3 variants, the same authors demonstrated that ivacaftor and genistein can rescue variants N568D, F629L, and G667R [ 81 ]. These observations make CFTR potentiators a potential therapeutic option for patients suffering from surfactant deficiency caused by ABCA3 variants. Other genes involved in surfactant production include SFTPA , SFTPB , and SFTPC , with variants within these genes leading to abnormal surfactant production and clearance [ 82 ]. Cyclosporine A (CsA), a calcineurin inhibitor, has recently been suggested as a new potential candidate for ABCA3-specific molecular correction using high-content screening [ 83 ] and was used in association with pirfenidone in a child suffering from systemic lupus erythematosus ILD. An improvement in symptoms, pulmonary function, and chest CT images was observed after 2 years of treatment [ 84 ]. Granulocyte-macrophage colony-stimulating factor (GM-CSF) is a cytokine with a key role in surfactant physiology, and GM-CSF −/− mice display defective clearance of surfactant by alveolar macrophages, leading to PAP [ 85 ]. The safety and efficacy of inhaled sargramostim, a recombinant human GM-CSF, were assessed in children with hereditary PAP caused by bi-allelic variants in CSF2RA or CSF2RB (NCT01511068). However, the study was prematurely discontinued because of slow recruitment. The effect of inhaled sargramostim in patients with PAP was also assessed in a phase 2, multicenter, randomized, double-blind, placebo-controlled trial conducted in Japan (NCT02835742). The study enrolled 64 patients, including patients aged 16–18 years [ 86 ]. The frequency and severity of the adverse events did not differ significantly between the sargramostim and placebo groups. The mean change in the alveolar–arterial oxygen gradient between baseline and week 25, the primary endpoint, was significantly better in the GM-CSF group than in the placebo group (− 4.50 ± 9.03 mmHg vs. 0.17 ± 10.50 mmHg; p = 0.02). However, inhaled GM-CSF provided no clinical benefits. On the other hand, in a more recent double-blind, placebo-controlled trial in adult patients with autoimmune PAP, molgramostim (an Escherichia coli -produced recombinant GM-CSF formulated as a nebulizer solution) resulted in greater improvements in pulmonary gas transfer and functional health status than placebo, with similar rates of adverse events [ 87 ]. Inhaled GM-CSF has also been used in pediatric patients with autoimmune PAP, among other treatments [ 88 ].
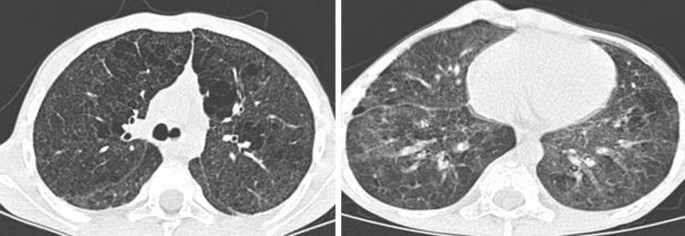
Seven-year-old boy ABCA3 -related interstitial lung disease. CT scans show bilateral ground glass opacity. CT computed tomography
Following anecdotal case reports [ 89 , 90 ], methionine has recently been evaluated in patients with PAP carrying pathogenic variants within MARS (NCT03887169) [ 91 ]. MARS encodes the methionyl–transfer RNA synthetase (MetRS), and the addition of methionine to the culture medium restores MetRS function in mutated yeast [ 92 ]. The study enrolled four children who were evaluated for respiratory, hepatic, and inflammation-related outcomes. For all patients, methionine supplementation was associated with respiratory improvement, reduced liver dysfunction, and reduced need for WLL. While encouraging, these data need to be validated in prospectively enrolled, larger populations of patients.
6.4 SAVI and COPA Disorders
STING-associated vasculopathy with onset in infancy (SAVI) is a genetic autoinflammatory disease secondary to perpetual STING (STimulator of INterferon Genes) activation. The disease, which is characterized by small vessel inflammation, generally has a neonatal or infantile-onset [ 93 ]. Recently, a phase 2/3 multicenter, open-label study (NCT04517253) [ 94 ] investigated the role of baricitinib, a Janus kinase (JAK)-1/2 inhibitor, in adult and pediatric Japanese patients with SAVI and other diseases, such as Nakajo–Nishimura syndrome (NNS) and Aicardi-Goutières syndrome (AGS). Nine patients were enrolled, including three with SAVI. At the end of the maintenance period (52 weeks), all patients experienced an improvement in their symptoms, and one patient reported a serious drug-related adverse event. COPA syndrome is a rare, genetic autoimmune disorder that is caused by dysfunctional coatomer associate protein subunit alpha (COPα), a protein that functions in the retrograde transport from the Golgi to the endoplasmic reticulum [ 39 ]. COPA syndrome can affect multiple organs, especially the lungs, joints, and kidneys [ 39 ]. Recent data have linked COPA mutations to STING-dependent interferon signaling. The JAK inhibitors baricitinib and ruxolitinib have recently been suggested as promising therapeutic options for patients with COPA syndrome, but additional data are needed to corroborate these preliminary findings [ 95 , 96 , 97 ].
7 Emerging Therapies
7.1 gene transfer therapies.
Gene transfer may allow the application of recent advances in molecular biology to clinical practice [ 98 ]. Synthetic gene transfer vectors have been tested in experimental models both in vitro and in vivo in patients with chILDs, including those related to dysfunctional SFTPC , SFTPB , and ABCA3 . Viral vectors (i.e., retroviral vectors, lentiviral vectors, adeno-associated viral [AAV] vectors, or adenoviral [Ad]-based vectors) have shown great potential in gene modulation, particularly for SP-B deficiency [ 99 , 100 , 101 ]. Nuclease-encoding, chemically modified mRNA is able to deliver site-specific nucleases in a mouse model of SP-B deficiency and improve survival of the animals. Kang and co-workers have recently shown that an AAV vector can restore surfactant activity and improve survival in SP-B knockout mice [ 102 ]. However, the development of a delivery vector requires knowledge of the precise disease target(s), transgene expression, and vector design. For instance, Ad-based vectors provide robust expression and a relatively large carrying capacity (~ 10 kb). Conversely, lentiviral vectors have a packaging capacity of at least 7.5 kb. Moreover, Ad-based vectors transduce both dividing and non-dividing cells with good tissue tropism and flexibility. Viral vectors have been evaluated in a range of diseases, including hereditary PAP with CSF2RA mutations. Hetzel and colleagues have shown that a lentiviral vector was able to induce Csf2ra complementary DNA (cDNA) expression in Csf2ra −/− macrophages, leading to restoration of GM-CSF signaling in hereditary PAP macrophages. The lentiviral vector had no adverse effects in the intended target cells, supporting testing lentivirus-mediated gene transfer therapy in hereditary PAP in humans [ 103 ].
7.2 Mesenchymal Stromal Cells
Cell therapy has fueled significant interest as a treatment for a range of respiratory diseases. Due to their low immunogenicity, easy isolation, and fleet differentiation in multiple lineages, mesenchymal stem cells (MSCs) are attractive therapeutic strategies also for chILD [ 104 ]. MSCs can be easily obtained from various fonts, including amniotic fluid, bone marrow, skeletal muscle, spleen, and lung. Previous studies in vitro and in vivo have explored the ability of MSCs to differentiate into alveolar epithelial cells, with the aim of assessing their potential utility in human lung disease [ 105 ]. Ahn and colleagues [ 106 ] conducted a phase 2, double-blind, placebo-controlled trial to assess the efficacy of intratracheal transplantation of human umbilical cord blood-derived MSCs (hUC-MSCs) (NCT01828957) in patients with BPD, a chronic lung disease limited to infants, typically caused by prolonged ventilation [ 107 ]. The study enrolled 66 premature infants aged between 23 and 28 gestational weeks. After 1 week, hUCB-MSC therapy significantly reduced the levels of several pro-inflammatory cytokines (i.e., interleukin [IL]-1, IL-6, IL-8, tumor necrosis factor [TNF]α, and matrix metallopeptidase [MMP]-9) in the tracheal aspirate fluid compared to placebo. However, the primary outcomes of survival and disease progression were not significantly improved by MSC transplantation. Based on a subgroup analysis suggesting that the secondary outcome of severe BPD was significantly improved in the 23–24 gestational week group, a larger phase 2 study is underway, focusing on infants in this age range (NCT03392467 – PNEUMOSTEM). Several clinical trials are currently evaluating the safety and efficacy of MSCs in BPD (ClinicalTrials.gov). MSCs are administered either intratracheally or intravenously. Induced pluripotent stem cells have been used in a mouse model of PAP induced by CSF2RB deficiency [ 108 ]. In addition, Wu and colleagues have shown that hUC-MSCs combined with low-dose pirfenidone reduce bleomycin-induced pulmonary fibrosis in mice more than the two therapies individually [ 109 ]. As with other therapeutic approaches, the safety and efficacy of MSCs in chILD need to be validated in larger studies.
7.3 Future Perspectives
In the past few years, genetic testing and whole genome sequencing (WGS) have increased both our ability to diagnose chILD and our understanding of disease pathobiology [ 110 ]. New insights have also emerged regarding disease-associated biomarkers. For example, unique protein signatures are shown in the bronchoalveolar lavage fluid (BALF) of NEHI patients and in other surfactant disorders [ 111 ]. Moreover, a number of blood biomarkers deeply investigated in adult ILD, including mucin-5B (MUC-5B) and Krebs von den Lungen-6 (KL-6), could also be useful in chILD to predict the risk of disease development and progression [ 112 , 113 ]. However, research focused on gene-to-protein translation is also needed. Because of the limited availability of human lung tissue (especially in children) and with animal models of lung fibrosis recapitulating only partially the complexity of human disease, lung “organoids” of varying cellular components have recently emerged as novel strategies to model, among other organs, the lung and airway [ 114 ]. Organoids are promising tools for studying complex cellular interactions, thus mimicking disease environments; indeed, they arise from colonies generated by single cells and maintain the genomic profile of the parent tissue. However, current organoid models cannot reproduce in toto the physiological repertoire of their respective organs [ 114 ].
8 Conclusion
The interest in childhood ILD has increased substantially in recent years, fueled mainly by genetic discoveries and the development of large national and international registries collecting rare and ultra-rare cases (Europe: chILD-EU; France: Respirare; USA: children; Australia: chILDRN). These consortia have also disseminated new knowledge on the management of these diseases and have improved the care for these children. Controlled studies of accepted but unproven treatments and novel treatments are urgently needed. To this end, participation of children in adult drug evaluation programs, the obligate implementation of pediatric investigational plans for all drugs introduced in adults, and the set-up of trials for conditions mainly prevalent in pediatrics must be realized. Continuing the build-up of international collaborations between expert centers and large databases of phenotypically well-defined patients is instrumental to any progress in these rare conditions.
Seidl E, Schwerk N, Carlens J, Wetzke M, Cunningham S, Emiralioğlu N, Kiper N, Lange J, Krenke K, Ullmann N, Krikovszky D, chILD-EU collaborators, Maqhuzu P, Griese CA, Schwarzkopf L, Griese M. Healthcare resource utilisation and medical costs for children with interstitial lung diseases (chILD) in Europe. Thorax. 2022;77(8):781–9. https://doi.org/10.1136/thoraxjnl-2021-217751 .
Article PubMed Google Scholar
Niemitz M, Schwerk N, Goldbeck L, Griese M. Development and validation of a health-related quality of life questionnaire for pediatric patients with interstitial lung disease. Pediatr Pulmonol. 2018;53(7):954–63. https://doi.org/10.1002/ppul.24018 .
Young L, et al. A national registry for childhood interstitial and diffuse lung diseases in the United States. (2018).
Griese M, Seidl E, Hengst M, Reu S, Rock H, Anthony G, Kiper N, Emiralioğlu N, Snijders D, Goldbeck L, Leidl R, Ley-Zaporozhan J, Krüger-Stollfuss I, Kammer B, Wesselak T, Eismann C, Schams A, Neuner D, MacLean M, Nicholson AG, Lauren M, Clement A, Epaud R, de Blic J, Ashworth M, Aurora P, Calder A, Wetzke M, Kappler M, Cunningham S, Schwerk N, Bush A, the other chILD-EU collaborators. International management platform for children’s interstitial lung disease (chILD-EU). Thorax. 2018;73(3):231–9. https://doi.org/10.1136/thoraxjnl-2017-210519 .
Casamento K, et al. Assessing the feasibility of a web-based registry for multiple orphan lung diseases: the Australasian Registry Network for Orphan Lung Disease (ARNOLD) experience. Orphanet J Rare Dis. 2016;11:1–6.
Article Google Scholar
Torrent-Vernetta A, Gaboli M, Castillo-Corullón S, Mondéjar-López P, Sanz Santiago V, Costa-Colomer J, Osona B, Torres-Borrego J, de la Serna-Blázquez O, Bellón Alonso S, Caro Aguilera P, Gimeno-DíazdeAtauri Á, Valenzuela Soria A, Ayats R, Martinde Vicente C, Velasco González V, Moure González JD, Canino Calderín EM, Pastor-Vivero MD, Villar Álvarez MÁ, Rovira-Amigo S, Iglesias Serrano I, Díez Izquierdo A, de MirMessa I, Gartner S, Navarro A, Baz-Redón N, Carmona R, Camats-Tarruella N, Fernández-Cancio M, Rapp C, Dopazo J, Griese M, Moreno-Galdó A, ChILD-Spain Group. Incidence and prevalence of children’s diffuse lung disease in Spain. Arch Bronconeumol. 2022;58(1):22–9. https://doi.org/10.1016/j.arbres.2021.06.001 . ( English, Spanish ).
Griese M. Chronic interstitial lung disease in children. Eur Respir Rev. 2018;27(147): 170100. https://doi.org/10.1183/16000617.0100-2017 .
Article PubMed PubMed Central Google Scholar
Nathan N, Griese M, Michel K, Carlens J, Gilbert C, Emiralioglu N, Torrent-Vernetta A, Marczak H, Willemse B, Delestrain C, Epaud R, ERS CRC chILD-EU group. Diagnostic workup of childhood interstitial lung disease. Eur Respir Rev. 2023;32(167):220188. https://doi.org/10.1183/16000617.0188-2022 .
Cunningham S, Jaffe A, Young LR. Children’s interstitial and diffuse lung disease. Lancet Child Adolesc Health. 2019;3(8):568–77. https://doi.org/10.1016/S2352-4642(19)30117-8 . ( Epub 2019 Jun 18 ).
Clement A, Nathan N, Epaud R, et al. Interstitial lung diseases in children. Orphanet J Rare Dis. 2010;5:22. https://doi.org/10.1186/1750-1172-5-22 .
Del Álamo M, Bührer C, Fisher D, Griese M, Lingor P, Palladini G, Sireau N, Hivert V, Sangiorgi L, Guillot F, Halftermeyer J, Soucková L, Nosková K, Demlová R. Identifying obstacles hindering the conduct of academic-sponsored trials for drug repurposing on rare-diseases: an analysis of six use cases. Trials. 2022;23(1):783. https://doi.org/10.1186/s13063-022-06713-y .
Ring AM, Schwerk N, Kiper N, Aslan AT, Aurora P, Ayats R, Azevedo I, Bandeira T, Carlens J, Castillo-Corullon S, Cobanoglu N, Elnazir B, Emiralioğlu N, Eyuboglu TS, Fayon M, Gursoy TR, Hogg C, Kötz K, Karadag B, Látalová V, Krenke K, Lange J, Manali ED, Osona B, Papiris S, Proesmann M, Reix P, Roditis L, Rubak S, Rumman N, Snijders D, Stehling F, Weiss L, Yalcın E, Zirek F, Bush A, Clement A, Griese M, Buchvald FF, Nathan N, Nielsen KG. Diffuse alveolar haemorrhage in children: an international multicentre study. ERJ Open Res. 2023;9(2):00733–2022. https://doi.org/10.1183/23120541.00733-2022 . ( Erratum in: ERJ Open Res. 2023 May 30;9(3) ).
Rauch D, Wetzke M, Reu S, Wesselak W, Schams A, Hengst M, Kammer B, Ley-Zaporozhan J, Kappler M, Proesmans M, Lange J, Escribano A, Kerem E, Ahrens F, Brasch F, Schwerk N, Griese M, PTI (Persistent Tachypnea of Infancy) Study Group of the Kids Lung Register. Persistent tachypnea of infancy. Usual and aberrant. Am J Respir Crit Care Med. 2016;193(4):438–47. https://doi.org/10.1164/rccm.201508-1655OC .
Clement A, ERS Task Force. Task force on chronic interstitial lung disease in immunocompetent children. Eur Respir J. 2004;24(4):686–97. https://doi.org/10.1183/09031936.04.00089803 .
Article CAS PubMed Google Scholar
Deutsch GH, Young LR, Deterding RR, Fan LL, Dell SD, Bean JA, Brody AS, Nogee LM, Trapnell BC, Langston C, Pathology Cooperative Group, ChILD Research Co-operative, et al. Diffuse lung disease in young children: application of a novel classification scheme. Am J Respir Crit Care Med. 2007;176:1120–8.
Kurland G, Deterding RR, Hagood JS, Young LR, Brody AS, Castile RG, Dell S, Fan LL, Hamvas A, Hilman BC, Langston C, Nogee LM, Redding GJ, American Thoracic Society Committee on Childhood Interstitial Lung Disease (chILD) and the chILD Research Network. An official American Thoracic Society clinical practice guideline: classification, evaluation, and management of childhood interstitial lung disease in infancy. Am J Respir Crit Care Med. 2013;188(3):376–94. https://doi.org/10.1164/rccm.201305-0923ST .
Bush A, Cunningham S, de Blic J, et al. European protocols for the diagnosis and initial treatment of interstitial lung disease in children. Thorax. 2015;70:1078–84.
Griese M. Etiologic classification of diffuse parenchymal (interstitial) lung diseases. J Clin Med. 2022;11:1747. https://doi.org/10.3390/jcm11061747 .
Article CAS PubMed PubMed Central Google Scholar
Koucký V, Pohunek P, Vašáková M, Bush A. Transition of patients with interstitial lung disease from paediatric to adult care. ERJ Open Res. 2021;7(2):00964–2020. https://doi.org/10.1183/23120541.00964-2020 .
Dervaux M, Thumerelle C, Fabre C, Abou-Taam R, Bihouee T, Brouard J, Clement A, Delacourt C, Delestrain C, Epaud R, Ghdifan S, Hadchouel A, Houdouin V, Labouret G, Perisson C, Reix P, Renoux MC, Troussier F, Weiss L, Mazenq J, Nathan N, Dubus JC. Long-term evolution of neuroendocrine cell hyperplasia of infancy: the FRENCHI findings. Eur J Pediatr. 2023;182(2):949–56.
Seidl E, Carlens J, Schwerk N, Wetzke M, Marczak H, Lange J, Krenke K, Mayell SJ, Escribano A, Seidenberg J, Ahrens F, Hebestreit H, Nährlich L, Sismanlar T, Aslan AT, Snijders D, Ullmann N, Kappler M, Griese M. Persistent tachypnea of infancy: follow up at school age. Pediatr Pulmonol. 2020;55(11):3119–25. https://doi.org/10.1002/ppul.25004 . ( Epub 2020 Aug 13 ).
Singh J, Jaffe A, Schultz A, Selvadurai H. Surfactant protein disorders in childhood interstitial lung disease. Eur J Pediatr. 2021;180(9):2711–21.
Trapnell BC, Nakata K, Bonella F, Campo I, Griese M, Hamilton J, Wang T, Morgan C, Cottin V, McCarthy C. Pulmonary alveolar proteinosis. Nat Rev Dis Primers. 2019;5(1):16.
Li Y, Seidl E, Knoflach K, Gothe F, Forstner ME, Michel K, Pawlita I, Gesenhues F, Sattler F, Yang X, Kroener C, Reu-Hofer S, Ley-Zaporozhan J, Kammer B, Krüger-Stollfuß I, Dinkel J, Carlens J, Wetzke M, Moreno-Galdó A, Torrent-Vernetta A, Lange J, Krenke K, Rumman N, Mayell S, Sismanlar T, Aslan A, Regamey N, Proesmans M, Stehling F, Naehrlich L, Ayse K, Becker S, Koerner-Rettberg C, Plattner E, Manali ED, Papiris SA, Campo I, Kappler M, Schwerk N, Griese M. ABCA3-related interstitial lung disease beyond infancy. Thorax. 2023;78(6):587–95. https://doi.org/10.1136/thorax-2022-219434 .
Manali ED, Legendre M, Nathan N, Kannengiesser C, Coulomb-L’Hermine A, Tsiligiannis T, Tomos P, Griese M, Borie R, Clement A, Amselem S, Crestani B, Papiris SA. Bi-allelic missense ABCA3 mutations in a patient with childhood ILD who reached adulthood. ERJ Open Res. 2019;5(3):00066–2019. https://doi.org/10.1183/23120541.00066-2019 .
van Moorsel CHM, van der Vis JJ, Grutters JC. Genetic disorders of the surfactant system: focus on adult disease. Eur Respir Rev. 2021;30(159): 200085. https://doi.org/10.1183/16000617.0085-2020 .
Nathan N, Sileo C, Calender A, Pacheco Y, Rosental PA, Cavalin C, Macchi O, Valeyre D, Clement A, French Sarcoidosis Group (GSF), Silicosis Research Group. Paediatric sarcoidosis. Paediatr Respir Rev. 2019;29:53–9.
PubMed Google Scholar
Tarvin SE, O’Neil KM. Systemic lupus erythematosus, Sjögren syndrome, and mixed connective tissue disease in children and adolescents. Pediatr Clin North Am. 2018;65(4):711–37.
Nevel RJ, Deutsch GH, Craven D, Deterding R, Fishman MP, Wambach JA, Casey A, Krone K, Liptzin DR, O'Connor MG, Kurland G, Taylor JB, Gower WA, Hagood JS, Conrad C, Tam-Williams JB, Fiorino EK, Goldfarb S, Sadreameli SC, Nogee LM, Montgomery G, Hamvas A, Laguna TA, Bansal M, Lew C, Santiago M, Popova A, De A, Chan M, Powers MR, Josephson MB, Camburn D, Voss L, Li Y, Young LR; chILD Registry Collaborative. The US national registry for childhood interstitial and diffuse lung disease: report of study design and initial enrollment cohort. Pediatr Pulmonol. 2023.
Nogee LM. Genetic basis of children’s interstitial lung disease. Pediatr Allergy Immunol Pulmonol. 2010;23:15–24.
Hamvas A. Inherited surfactant protein-B deficiency and surfactant protein-C associated disease: clinical features and evaluation. Semin Perinatol. 2006;30:316–26.
Doan M, Guillerman R, Dishop M, Nogee L, Langston C, Mallory G, Sockrider M, Fan L. Clinical, radiological and pathological features of ABCA3 mutations in children. Thorax. 2008;63:366–73.
Patel NJ, Jankovic J. NKX2-1-related disorders. 2014 [updated 2016 Jul 29]. In: Adam MP, Mirzaa GM, Pagon RA, Wallace SE, Bean LJH, Gripp KW, Amemiya A, editors. GeneReviews ® [Internet]. Seattle: University of Washington, Seattle; 1993–2023.
Kröner C, Reu S, Teusch V, Schams A, Grimmelt AC, Barker M, Brand J, Gappa M, Kitz R, Kramer BW, Lange L, Lau S, Pfannenstiel C, Proesmans M, Seidenberg J, Sismanlar T, Aslan AT, Werner C, Zielen S, Zarbock R, Brasch F, Lohse P, Griese M. Genotype alone does not predict the clinical course of SFTPC deficiency in paediatric patients. Eur Respir J. 2015;46(1):197–206. https://doi.org/10.1183/09031936.00129414 .
Kröner C, Wittmann T, Reu S, Teusch V, Klemme M, Rauch D, Hengst M, Kappler M, Cobanoglu N, Sismanlar T, Aslan AT, Campo I, Proesmans M, Schaible T, Terheggen-Lagro S, Regamey N, Eber E, Seidenberg J, Schwerk N, Aslanidis C, Lohse P, Brasch F, Zarbock R, Griese M. Lung disease caused by ABCA3 mutations. Thorax. 2017;72(3):213–20. https://doi.org/10.1136/thoraxjnl-2016-208649 .
Hildebrandt J, Yalcin E, Bresser HG, Cinel G, Gappa M, Haghighi A, Kiper N, Khalilzadeh S, Reiter K, Sayer J, Schwerk N, Sibbersen A, Van Daele S, Nübling G, Lohse P, Griese M. Characterization of CSF2RA mutation related juvenile pulmonary alveolar proteinosis. Orphanet J Rare Dis. 2014;26(9):171.
Suzuki T, Maranda B, Sakagami T, Catellier P, Couture CY, Carey BC, Chalk C, Trapnell BC. Hereditary pulmonary alveolar proteinosis caused by recessive CSF2RB mutations. Eur Respir J. 2011;37(1):201–4. https://doi.org/10.1183/09031936.00090610 .
Gao X, Michel K, Griese M. Interstitial lung disease in immunocompromised children. Diagnostics (Basel). 2022;13(1):64. https://doi.org/10.3390/diagnostics13010064 .
Kumrah R, Mathew B, Vignesh P, Singh S, Rawat A. Genetics of COPA syndrome. Appl Clin Genet. 2019;8(12):11–8.
Galambos C, Mullen MP, Shieh JT, Schwerk N, Kielt MJ, Ullmann N, Boldrini R, Stucin-Gantar I, Haass C, Bansal M, Agrawal PB, Johnson J, Peca D, Surace C, Cutrera R, Pauciulo MW, Nichols WC, Griese M, Ivy D, Abman SH, Austin ED, Danhaive O. Phenotype characterisation of TBX4 mutation and deletion carriers with neonatal and paediatric pulmonary hypertension. Eur Respir J. 2019;54(2):1801965. https://doi.org/10.1183/13993003.01965-2018 .
Meliota G, Vairo U, Ficarella R, Milella L, Faienza MF, D’Amato G. Cardiovascular, brain, and lung involvement in a newborn with a novel FLNA mutation: a case report and literature review. Adv Neonatal Care. 2022;22(2):125–31.
Yurdakök M. Inherited disorders of neonatal lung diseases. Turk J Pediatr. 2004;46(2):105–14.
Liptzin DR, Pickett K, Brinton JT, Agarwal A, Fishman MP, Casey A, Towe CT, Taylor JB, Kurland G, Hagood JS, Wambach J, Srivastava R, Al-Saleh H, Dell SD, Young LR, Deterding RR. Neuroendocrine cell hyperplasia of infancy. Clinical score and comorbidities. Ann Am Thorac Soc. 2020;17(6):724–8.
Dinwiddie R, Sharief N, Crawford O. Idiopathic interstitial pneumonitis in children: a national survey in the United Kingdom and Ireland. Pediatr Pulmonol. 2002;34(1):23–9. https://doi.org/10.1002/ppul.10125 .
Hines EJ, Walsh M, Armes JE, Douglas T, Chawla J. Interstitial lung disease in infancy: a general approach. J Paediatr Child Health. 2016;52:370–6.
Seidl E, Schwerk N, Carlens J, Wetzke M, Emiralioğlu N, Kiper N, Lange J, Krenke K, Szepfalusi Z, Stehling F, Baden W, Hämmerling S, Jerkic SP, Proesmans M, Ullmann N, Buchvald F, Knoflach K, Kappler M, chILD EU collaborators, Griese M. Acute exacerbations in children’s interstitial lung disease. Thorax. 2022;77(8):799–804. https://doi.org/10.1136/thoraxjnl-2021-217941 .
Desmarquest P, Tamalet A, Fauroux B, Boule M, Boccon-Gibod L, Tournier G, Clement A. Chronic interstitial lung disease in children: response to high-dose intravenous methylprednisolone pulses. Pediatr Pulmonol. 1998;26(5):332–8. https://doi.org/10.1002/(sici)1099-0496(199811)26:5%3c332::aid-ppul5%3e3.0.co;2-q .
de Benedictis FM, Bush A. Corticosteroids in respiratory diseases in children. Am J Respir Crit Care Med. 2012;185(1):12–23.
Breuer O, Schultz A. Side effects of medications used to treat childhood interstitial lung disease. Paediatr Respir Rev. 2018;28:68–79.
Rainsford KD, Parke AL, Clifford-Rashotte M, et al. Therapy and pharmacological properties of hydroxychloroquine and chloroquine in treatment of systemic lupus erythematosus, rheumatoid arthritis and related diseases. Inflammopharmacology. 2015;23:231–69. https://doi.org/10.1007/s10787-015-0239-y .
Braun S, Ferner M, Kronfeld K, Griese M. Hydroxychloroquine in children with interstitial (diffuse parenchymal) lung diseases. Pediatr Pulmonol. 2015;50(4):410–9.
Modjtahedi BS, Movassagh N, Gandhi N, Morse LS, Maibach HI. Screening for hydroxychloroquine toxicity in children. Cutan Ocul Toxicol. 2013;32(4):344.
Griese M, Köhler M, Witt S, Sebah D, Kappler M, Wetzke M, Schwerk N, Emiralioglu N, Kiper N, Kronfeld K, et al. Prospective evaluation of hydroxychloroquine in pediatric interstitial lung diseases: Study protocol for an investigator-initiated, randomized controlled, parallel-group clinical trial. Trials. 2020;21:307.
Griese M, Kappler M, Stehling F, Schulze J, Baden W, Koerner-Rettberg C, Carlens J, Prenzel F, Nährlich L, Thalmeier A, Sebah D, Kronfeld K, Rock H, Ruckes C, HCQ-study group, Wetzke M, Seidl E, Schwerk N. Randomized controlled phase 2 trial of hydroxychloroquine in childhood interstitial lung disease. Orphanet J Rare Dis. 2022;17(1):289.
Yang X, Forstner M, Rapp CK, Rothenaigner I, Li Y, Hadian K, Griese M. ABCA3 deficiency-variant-specific response to hydroxychloroquine. Int J Mol Sci. 2023;24(9):8179. https://doi.org/10.3390/ijms24098179 .
Thouvenin G, Nathan N, Epaud R, Clement A. Diffuse parenchymal lung disease caused by surfactant deficiency: dramatic improvement by azithromycin. BMJ Case Rep. 2013;2013:bcr2013009988.
Bush A. Azithromycin is the answer in paediatric respiratory medicine, but what was the question? Paediatr Respir Rev. 2020;34:67–74.
Klay D, Grutters JC, van der Vis JJ, Platenburg MGJP, Kelder JC, Tromp E, van Moorsel CHM. Progressive disease with low survival in adult patients with pulmonary fibrosis carrying surfactant-related gene mutations: an observational study. Chest. 2023;163(4):870–80. https://doi.org/10.1016/j.chest.2022.11.002 .
Reiter K, Schoen C, Griese M, Nicolai T. Whole-lung lavage in infants and children with pulmonary alveolar proteinosis. Paediatr Anaesth. 2010;20(12):1118–23. https://doi.org/10.1111/j.1460-9592.2010.03442.x .
Paschen C, Reiter K, Stanzel F, Teschler H, Griese M. Therapeutic lung lavages in children and adults. Respir Res. 2005;6(1):138. https://doi.org/10.1186/1465-9921-6-138 .
Bush A, Pabary R. Pulmonary alveolarproteinosis in children. Breathe (Sheff). 2020;16(2): 200001. https://doi.org/10.1183/20734735.0001-2020 .
Wilson CA, Wilmshurst SL, Black AE. Anesthetic techniques to facilitate lung lavage for pulmonary alveolar proteinosis in children-new airway techniques and a review of the literature. Paediatr Anaesth. 2015;25(6):546–53.
Seidl E, Schramm D, Schön C, Reiter K, Pawlita I, Kappler M, Reu-Hofer S, Hauck F, Albert M, Griese M. Pulmonary alveolar proteinosis due to heterozygous mutation in OAS1: whole lung lavages for long-term bridging to hematopoietic stem cell transplantation. Pediatr Pulmonol. 2022;57(1):273–7. https://doi.org/10.1002/ppul.25728 .
Griese M, Zarbock R, Costabel U, Hildebrandt J, Theegarten D, Albert M, Thiel A, Schams A, Lange J, Krenke K, Wesselak T, Schön C, Kappler M, Blum H, Krebs S, Jung A, Kröner C, Klein C, Campo I, Luisetti M, Bonella F. GATA2 deficiency in children and adults with severe pulmonary alveolar proteinosis and hematologic disorders. BMC Pulm Med. 2015;12(15):87. https://doi.org/10.1186/s12890-015-0083-2 .
Article CAS Google Scholar
Khirani S, Nathan N, Ramirez A, Aloui S, Delacourt C, Clément A, Fauroux B. Work of breathing in children with diffuse parenchymal lung disease. Respir Physiol Neurobiol. 2015;15(206):45–52.
Dziekiewicz MA, Karolewska-Bochenek K, Dembiński Ł, Gawronska A, Krenke K, Lange J, Banasiuk M, Kuchar E, Kulus M, Albrecht P, Banaszkiewicz A. Gastroesophageal reflux disease in children with interstitial lung disease. Adv Exp Med Biol. 2016;912:57–64.
Iablonskii P, Carlens J, Mueller C, Aburahma K, Niehaus A, Boethig D, Franz M, Floethmann K, Sommer W, Optenhoefel J, Tudorache I, Greer M, Koeditz H, Jack T, Hansmann G, Kuehn C, Horke A, Hansen G, Haverich A, Warnecke G, Avsar M, Salman J, Bobylev D, Ius F, Schwerk N. Indications and outcome after lung transplantation in children under 12 years of age: a 16-year single center experience. J Heart Lung Transplant. 2022;41(2):226–36.
Kumata S, Matsuda Y, Oishi H, Sado T, Niikawa H, Watanabe T, Noda M, Hoshikawa Y, Sakurada A, Saito-Koyama R, Niizuma H, Kitazawa H, Akiba M, Sasahara Y, Okada Y. Living donor lobar lung transplant for a patient with lung disease caused by ABCA3 gene mutations: a case report. Transplant Proc. 2022;54(10):2803–6. https://doi.org/10.1016/j.transproceed.2022.07.020 .
Hime NJ, Zurynski Y, Fitzgerald D, Selvadurai H, Phu A, Deverell M, Elliott EJ, Jaffe A. Childhood interstitial lung disease: a systematic review. Pediatr Pulmonol. 2015;50(12):1383–92. https://doi.org/10.1002/ppul.23183 . ( Epub 2015 Apr 30 ).
Deterding RR, DeBoer EM, Cidon MJ, Robinson TE, Warburton D, Deutsch GH, Young LR. Approaching clinical trials in childhood interstitial lung disease and pediatric pulmonary fibrosis. Am J Respir Crit Care Med. 2019;200(10):1219–27. https://doi.org/10.1164/rccm.201903-0544CI .
King TE Jr, Bradford WZ, Castro-Bernardini S, Fagan EA, Glaspole I, Glassberg MK, Gorina E, Hopkins PM, Kardatzke D, Lancaster L, Lederer DJ, Nathan SD, Pereira CA, Sahn SA, Sussman R, Swigris JJ, Noble PW, ASCEND Study Group. A phase 3 trial of pirfenidone in patients with idiopathic pulmonary fibrosis. N Engl J Med. 2014;370(22):2083–92. https://doi.org/10.1056/NEJMoa1402582 . ( Epub 2014 May 18. Erratum in: N Engl J Med. 2014 Sep 18;371(12):1172 ).
Richeldi L, du Bois RM, Raghu G, Azuma A, Brown KK, Costabel U, Cottin V, Flaherty KR, Hansell DM, Inoue Y, Kim DS, Kolb M, Nicholson AG, Noble PW, Selman M, Taniguchi H, Brun M, Le Maulf F, Girard M, Stowasser S, Schlenker-Herceg R, Disse B, Collard HR, INPULSIS Trial Investigators. Efficacy and safety of nintedanib in idiopathic pulmonary fibrosis. N Engl J Med. 2014;370(22):2071–82. https://doi.org/10.1056/NEJMoa1402584 . ( Epub 2014 May 18. Erratum in: N Engl J Med. 2015 Aug 20;373(8):782 ).
Flaherty KR, Wells AU, Cottin V, Devaraj A, Walsh SLF, Inoue Y, Richeldi L, Kolb M, Tetzlaff K, Stowasser S, Coeck C, Clerisme-Beaty E, Rosenstock B, Quaresma M, Haeufel T, Goeldner RG, Schlenker-Herceg R, Brown KK, INBUILD Trial Investigators. Nintedanib in progressive fibrosing interstitial lung diseases. N Engl J Med. 2019;381(18):1718–27. https://doi.org/10.1056/NEJMoa1908681 .
Deterding R, Young LR, DeBoer EM, Warburton D, Cunningham S, Schwerk N, Flaherty KR, Brown KK, Dumistracel M, Erhardt E, Bertulis J, Gahlemann M, Stowasser S, Griese M, InPedILD trial investigators. Nintedanib in children and adolescents with fibrosing interstitial lung diseases. Eur Respir J. 2023;61(2):2201512. https://doi.org/10.1183/13993003.01512-2022 .
Gozal D, Kolb M. Nintedanib in chILD: a small step, yes… but at least a step forward in a marathon! Eur Respir J. 2023;61(2):2201797. https://doi.org/10.1183/13993003.01797-2022 .
Armanios M, Blackburn EH. The telomere syndromes. Nat Rev Genet. 2012;13(10):693–704. https://doi.org/10.1038/nrg3246 . ( Epub 2012 Sep 11. Erratum in: Nat Rev Genet. 2013 Mar;14(3):235 ).
Savage SA, Alter BP. Dyskeratosis congenita. Hematol Oncol Clin North Am. 2009;23:215–31.
Townsley DM, Dumitriu B, Liu D, Biancotto A, Weinstein B, Chen C, Hardy N, Mihalek AD, Lingala S, Kim YJ, Yao J, Jones E, Gochuico BR, Heller T, Wu CO, Calado RT, Scheinberg P, Young NS. Danazol treatment for telomere diseases. N Engl J Med. 2016;374(20):1922–31. https://doi.org/10.1056/NEJMoa1515319 .
Mackintosh JA, Pietsch M, Lutzky V, Enever D, Bancroft S, Apte SH, Tan M, Yerkovich ST, Dickinson JL, Pickett HA, Selvadurai H, Grainge C, Goh NS, Hopkins P, Glaspole I, Reynolds PN, Wrobel J, Jaffe A, Corte TJ, Chambers DC. TELO-SCOPE study: a randomised, double-blind, placebo-controlled, phase 2 trial of danazol for short telomere related pulmonary fibrosis. BMJ Open Respir Res. 2021;8(1): e001127. https://doi.org/10.1136/bmjresp-2021-001127 .
Kinting S, Höppner S, Schindlbeck U, Forstner ME, Harfst J, Wittmann T, Griese M. Functional rescue of misfolding ABCA3 mutations by small molecular correctors. Hum Mol Genet. 2018;27(6):943–53.
Kinting S, Li Y, Forstner M, Delhommel F, Sattler M, Griese M. Potentiation of ABCA3 lipid transport function by ivacaftor and genistein. J Cell Mol Med. 2019;23(8):5225–34. https://doi.org/10.1111/jcmm.14397 .
Whitsett JA, Wert SE, Weaver TE. Alveolar surfactant homeostasis and the pathogenesis of pulmonary disease. Annu Rev Med. 2010;61:105–19.
Forstner M, Lin S, Yang X, Kinting S, Rothenaigner I, Schorpp K, Li Y, Hadian K, Griese M. High-content screening identifies cyclosporin A as a novel ABCA3-specific molecular corrector. Am J Respir Cell Mol Biol. 2022;66(4):382–90. https://doi.org/10.1165/rcmb.2021-0223OC .
Deng L, Chen Y, Hu X, Zhou J, Zhang Y. Case report: successful treatment of refractory interstitial lung disease with cyclosporine A and pirfenidone in a child with SLE. Front Immunol. 2021;4(12): 708463. https://doi.org/10.3389/fimmu.2021.708463 .
Bonella F, Borie R. Targeted therapy for pulmonary alveolar proteinosis: the time is now. Eur Respir J. 2022;59(4):2102971. https://doi.org/10.1183/13993003.02971-2021 .
Tazawa R, Ueda T, Abe M, Tatsumi K, Eda R, Kondoh S, Morimoto K, Tanaka T, Yamaguchi E, Takahashi A, Oda M, Ishii H, Izumi S, Sugiyama H, Nakagawa A, Tomii K, Suzuki M, Konno S, Ohkouchi S, Tode N, Handa T, Hirai T, Inoue Y, Arai T, Asakawa K, Sakagami T, Hashimoto A, Tanaka T, Takada T, Mikami A, Kitamura N, Nakata K. Inhaled GM-CSF for pulmonary alveolar proteinosis. N Engl J Med. 2019;381(10):923–32. https://doi.org/10.1056/NEJMoa1816216 .
Trapnell BC, Inoue Y, Bonella F, Morgan C, Jouneau S, Bendstrup E, Campo I, Papiris SA, Yamaguchi E, Cetinkaya E, Ilkovich MM, Kramer MR, Veltkamp M, Kreuter M, Baba T, Ganslandt C, Tarnow I, Waterer G, Jouhikainen T, IMPALA Trial Investigators. Inhaled molgramostim therapy in autoimmune pulmonary alveolar proteinosis. N Engl J Med. 2020;383(17):1635–44. https://doi.org/10.1056/NEJMoa1913590 .
Griese M, Panagiotou P, Manali ED, Stahl M, Schwerk N, Costa V, Douros K, Kallieri M, Urbantat RM, von Bernuth H, Kolilekas L, Morais L, Ramos A, Landwehr K, Knoflach K, Gothe F, Reiter K, Papaevangelou V, Kaditis AG, Kanaka-Gantenbein C, Papiris SA. Autoimmune pulmonary alveolar proteinosis in children. ERJ Open Res. 2022;8(1):00701–2021. https://doi.org/10.1183/23120541.00701-2021 . ( eCollection 2022 Jan ).
Lenz D, Stahl M, Seidl E, Schöndorf D, Brennenstuhl H, Gesenhues F, Heinzmann T, Longerich T, Mendes MI, Prokisch H, Salomons GS, Schön C, Smith DEC, Sommerburg O, Wagner M, Westhoff JH, Reiter K, Staufner C, Griese M. Rescue of respiratory failure in pulmonary alveolar proteinosis due to pathogenic MARS1 variants. Pediatr Pulmonol. 2020;55(11):3057–66. https://doi.org/10.1002/ppul.25031 . ( Epub 2020 Sep 7 ).
Rips J, Meyer-Schuman R, Breuer O, et al. MARS variant associated with both recessive interstitial lung and liver disease and dominant Charcot–Marie–Tooth disease. Eur J Med Genet. 2018;61:616–20. https://doi.org/10.1016/j.ejmg.2018.04.005 .
Hadchouel A, Drummond D, Pontoizeau C, Aoust L, Hurtado Nedelec MM, El Benna J, Gachelin E, Perisson C, Vigier C, Schiff M, Lacaille F, Molina TJ, Berteloot L, Renolleau S, Ottolenghi C, Tréluyer JM, de Blic J, Delacourt C. Methionine supplementation for multi-organ dysfunction in MetRS-related pulmonary alveolar proteinosis. Eur Respir J. 2022;59(4):2101554. https://doi.org/10.1183/13993003.01554-2021 .
Hadchouel A, Wieland T, Griese M, Baruffini E, Lorenz-Depiereux B, Enaud L, Graf E, Dubus JC, Halioui-Louhaichi S, Coulomb A, et al. Biallelicmutations of methionyl-tRNA synthetase cause aspecific type of pulmonary alveolar proteinosisprevalent on Reunion island. Am J Hum Genet. 2015;96:826–31.
Liu Y, Jesus AA, Marrero B, Yang D, Ramsey SE, Sanchez GAM, Tenbrock K, Wittkowski H, Jones OY, Kuehn HS, Lee CR, DiMattia MA, Cowen EW, Gonzalez B, Palmer I, DiGiovanna JJ, Biancotto A, Kim H, Tsai WL, Trier AM, Huang Y, Stone DL, Hill S, Kim HJ, St Hilaire C, Gurprasad S, Plass N, Chapelle D, Horkayne-Szakaly I, Foell D, Barysenka A, Candotti F, Holland SM, Hughes JD, Mehmet H, Issekutz AC, Raffeld M, McElwee J, Fontana JR, Minniti CP, Moir S, Kastner DL, Gadina M, Steven AC, Wingfield PT, Brooks SR, Rosenzweig SD, Fleisher TA, Deng Z, Boehm M, Paller AS, Goldbach-Mansky R. Activated STING in a vascular and pulmonary syndrome. N Engl J Med. 2014;371(6):507–18.
Kanazawa N, Ishii T, Takita Y, Nishikawa A, Nishikomori R. Efficacy and safety of baricitinib in Japanese patients with autoinflammatory type I interferonopathies (NNS/CANDLE, SAVI, And AGS). Pediatr Rheumatol Online J. 2023;21(1):38.
Krutzke S, Rietschel C, Horneff G. Baricitinib in therapy of COPA syndrome in a 15-year-old girl. Eur J Rheumatol. 2020;7(Suppl1):S78–81.
Frémond ML, Nathan N. COPA syndrome, 5 years after: where are we? Jt Bone Spine. 2021;88(2): 105070.
Frémond ML, Legendre M, Fayon M, Clement A, Filhol-Blin E, Richard N, Berdah L, Roullaud S, Rice GI, Bondet V, Duffy D, Sileo C, Ducou le Pointe H, Begueret H, Coulomb A, Neven B, Amselem S, Crow Y, Nathan N. Use of ruxolitinib in COPA syndrome manifesting as life-threatening alveolar haemorrhage. Thorax. 2020;75(1):92–5.
Pelizzo G, et al. Mesenchymal stromal cells for the treatment of interstitial lung disease in children: a look from pediatric and pediatric surgeon viewpoints. Cells. 2021;10(12):3270.
Mahiny AJ, Dewerth A, Mays LE, Alkhaled M, Mothes B, Malaeksefat E, Loretz B, Rottenberger J, Brosch DM, Reautschnig P, Surapolchai P, Zeyer F, Schams A, Carevic M, Bakele M, Griese M, Schwab M, Nürnberg B, Beer-Hammer S, Handgretinger R, Hartl D, Lehr CM, Kormann MS. In vivo genome editing using nuclease-encoding mRNA corrects SP-B deficiency. Nat Biotechnol. 2015;33(6):584–6. https://doi.org/10.1038/nbt.3241 . ( Epub 2015 May 18 ).
Cooney AL, Wambach JA, Sinn PL, McCray PB Jr. Gene therapy potential for genetic disorders of surfactant dysfunction. Front Genome Ed. 2022;14(3): 785829. https://doi.org/10.3389/fgeed.2021.785829 .
Presti S, Parisi GF, Papale M, Gitto E, Manti S, Leonardi S. Interstitial lung disease in children: “Specific Conditions of Undefined Etiology” becoming clearer. Children (Basel). 2022;9(11):1744. https://doi.org/10.3390/children9111744 .
Kang MH, van Lieshout LP, Xu L, Domm JM, Vadivel A, Renesme L, et al. A lung tropic AAV vector improves survival in a mouse model of surfactant B deficiency. Nat Commun. 2020;11:3929. https://doi.org/10.1038/s41467-020-17577-8 .
Hetzel M, et al. Function and safety of lentivirus-mediated gene transfer for CSF2RA-deficiency. Hum Gene Therapy Methods. 2017;28(6):318–29.
Brennan LC, O’Sullivan A, MacLoughlin R. Cellular therapy for the treatment of paediatric respiratory disease. Int J Mol Sci. 2021;22(16):8906.
Liu J, Peng D, You J, Zhou O, Qiu H, Hao C, Chen H, Fu Z, Zou L. Type 2 alveolar epithelial cells differentiated from human umbilical cord mesenchymal stem cells alleviate mouse pulmonary fibrosis through β-catenin-regulated cell apoptosis. Stem Cells Dev. 2021;30(13):660–70. https://doi.org/10.1089/scd.2020.0208 .
Ahn SY, Chang YS, Lee MH, Sung SI, Lee BS, Kim KS, Kim AR, Park WS. Stem cells for bronchopulmonary dysplasia in preterm infants: a randomized controlled phase II trial. Stem Cells Transl Med. 2021;10(8):1129–37. https://doi.org/10.1002/sctm.20-0330 .
Ehrenkranz RA, Walsh MC, Vohr BR, Jobe AH, Wright LL, Fanaroff AA, Wrage LA, Poole K, National Institutes of Child Health and Human Development Neonatal Research, Network. Validation of the National Institutes of Health consensus definition of bronchopulmonary dysplasia. Pediatrics. 2005;116(6):1353–60. https://doi.org/10.1542/peds.2005-0249 .
Mucci A, Lopez-Rodriguez E, Hetzel M, Liu S, Suzuki T, Happle C, Ackermann M, Kempf H, Hillje R, Kunkiel J, Janosz E, Brennig S, Glage S, Bankstahl JP, Dettmer S, Rodt T, Gohring G, Trapnell B, Hansen G, Trapnell C, Knudsen L, Lachmann N, Moritz T. iPSC-derived macrophages effectively treat pulmonary alveolar proteinosis in Csf2rb-deficient mice. Stem Cell Reports. 2018;11(3):696–710. https://doi.org/10.1016/j.stemcr.2018.07.006 .
Wu X, Gou H, Zhou O, Qiu H, Liu H, Fu Z, Chen L. Human umbilical cord mesenchymal stem cells combined with pirfenidone upregulates the expression of RGS2 in the pulmonary fibrosis in mice. Respir Res. 2022;23(1):270. https://doi.org/10.1186/s12931-022-02192-6 .
Vece TJ, Wambach JA, Hagood JS. Childhood rare lung disease in the 21st century: “-omics” technology advances accelerating discovery. Pediatr Pulmonol. 2020;55(7):1828–37.
Deterding RR, Wagner BD, Harris JK, DeBoer EM. Pulmonary aptamer signatures in children’s interstitial and diffuse lung diseases. Am J Respir Crit Care Med. 2019;200(12):1496–504.
Nathan N, Corvol H, Amselem S, Clement A. Biomarkers in interstitial lung diseases. Paediatr Respir Rev. 2015;16:219–24.
Kilinc AA, Arslan A, Yildiz M, Kucur M, Adrovic A, Barut K, Sahin S, Cokugras H, Kasapcopur O. Serum KL-6 level as a biomarker of interstitial lung disease in childhood connective tissue diseases: a pilot study. Rheumatol Int. 2020;40(10):1701–6.
Zhao Z, Chen X, Dowbaj AM, et al. Organoids. Nat Rev Methods Primers. 2022;2:94. https://doi.org/10.1038/s43586-022-00174-y .
Download references
Author information
Authors and affiliations.
Respiratory Disease Unit, Department of Cardiac Thoracic, Vascular Sciences and Public Health, University of Padova, Via N. Giustiniani n°2, 35128, Padua, Italy
Nicol Bernardinello & Paolo Spagnolo
Department of Pediatric Pneumology, Dr. von Hauner Children’s Hospital, German Center for Lung Research (DZL), Ludwig-Maximilians University, Munich, Germany
Matthias Griese
Université de Paris, INSERM UMR 1152, Service de Pneumologie A, Centre de compétences maladies pulmonaires rares, Hôpital Bichat-Claude Bernard, AP-HP, 75018, Paris, France
Raphaël Borie
You can also search for this author in PubMed Google Scholar
Corresponding author
Correspondence to Paolo Spagnolo .
Ethics declarations
Conflict of interest.
The authors declare no conflict of interest.
Author contributions
The authors contributed equally to the work. All authors have critically revised and approved the final version of the manuscript.
Availability of data
Not applicable.
Open access funding provided by Università degli Studi di Padova within the CRUI-CARE Agreement. No sources of financial assistance were used to conduct the study/analysis described in the article and/or used to assist with the preparation of the manuscript.
Consent to participate
Consent for publication, code availability, rights and permissions.
Open Access This article is licensed under a Creative Commons Attribution-NonCommercial 4.0 International License, which permits any non-commercial use, sharing, adaptation, distribution and reproduction in any medium or format, as long as you give appropriate credit to the original author(s) and the source, provide a link to the Creative Commons licence, and indicate if changes were made. The images or other third party material in this article are included in the article's Creative Commons licence, unless indicated otherwise in a credit line to the material. If material is not included in the article's Creative Commons licence and your intended use is not permitted by statutory regulation or exceeds the permitted use, you will need to obtain permission directly from the copyright holder. To view a copy of this licence, visit http://creativecommons.org/licenses/by-nc/4.0/ .
Reprints and permissions
About this article
Bernardinello, N., Griese, M., Borie, R. et al. Emerging Treatments for Childhood Interstitial Lung Disease. Pediatr Drugs 26 , 19–30 (2024). https://doi.org/10.1007/s40272-023-00603-9
Download citation
Accepted : 17 October 2023
Published : 10 November 2023
Issue Date : January 2024
DOI : https://doi.org/10.1007/s40272-023-00603-9
Share this article
Anyone you share the following link with will be able to read this content:
Sorry, a shareable link is not currently available for this article.
Provided by the Springer Nature SharedIt content-sharing initiative
- Find a journal
- Publish with us
- Track your research
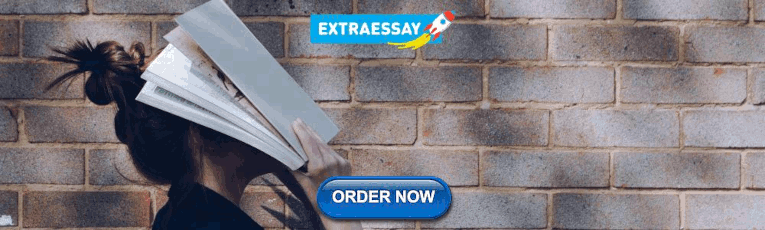
IMAGES
VIDEO
COMMENTS
This is no ordinary origami paper, it's made out of organ tissues and could eventually become a high-tech band aid. Northwestern University. When Adam Jakus was a postdoc at Northwestern ...
An important aspect of using human tissues in research is tissue quality. Bioresources use a number of approaches to assure high quality and fitness-for-purpose of the biospecimens they provide. This includes establishing a rigorous quality management system (QMS) with demanding quality control (QC).
Journal of Tissue Engineering. Journal of Tissue Engineering (JTE) is a peer-reviewed, open access journal which focuses on scientific research in the field of tissue engineering and its clinical application. View full journal description. This journal is a member of the Committee on Publication Ethics (COPE).
Overview. Cell and Tissue Research is a scholarly forum publishing articles in the fields of molecular, cell, and supracellular biology. Provides thorough coverage across the fields of molecular, cell, and supracellular biology. Emphasizes on structure-function relationships as revealed by recombinant molecular technologies.
We discuss the various types of bioresources that make human tissue available, and advise on how investigators can find and use appropriate bioresources to support their research — with the hope that this information will help facilitate the transition from research on animals to research using human tissues, as rapidly as is practicable.
The structure of the fibre network in tissue paper can be complex and difficult to analyze, due to the presence of superimposed structures such as creping and patterns that occur, for example, in through-air-dried (TAD) tissue. Properties like high absorbency, a pleasant handfeel, and strength-related characteristics are closely related to the fibre network structure. Therefore, in addition to ...
Global paper production has been steadily increasing over the years and reached approximately 425 million tons per year in 2020 (FAO—Forestry Production and Trade 2020).Hygiene tissue papers are one of the fastest-growing categories of all paper products witnessing a CAGR (compound annual growth rate) of 2.0% between 2015 and 2020 (FAO—Forestry Production and Trade 2020).
Tissue engineering is a set of methods that can replace or repair damaged or diseased tissues with natural, synthetic, or semisynthetic tissue mimics. These mimics can either be fully functional ...
Workshop participants were tasked with identifying the challenges of accessing and qualifying tissues for research purposes and creating a strategy to help meet the needs of the research communities to increase the availability and quality of human tissues in biomedical and translational research. Break-out groups identified significant ...
Tissue paper materials are lightweight creped papers used for cleaning, hygiene, and cosmetic purposes, including facial papers, toilet papers, towel papers, napkins, diapers, facial masks, among others. ... (ERDF) in the frame of COMPETE 2020 nº 246/AXIS II/2017 and by research unit Fiber Materials and Environmental Technologies ...
Linking Clinical Data to Biological Samples. An essential criterion for any modern biobank is the ability to link research findings derived from tissue samples to robust clinical and pathological data. Simply providing tissue or other biosamples is no longer sufficient for translational research. This is essential in biomarker studies, in order ...
The court found that the tissue was given voluntarily for research without any expectation of return, and therefore the plaintiffs had no ownership interest in the tissues, or the research performed using the tissue (7, 10). The court noted that a contrary rule would cripple medical research because it would "bestow a continuing right for ...
The paper underlines how reaction to implant of PLA and its copolymer is always local and close to the implanted material and not systemic in nature . 5.1. Synthesis and Properties. ... Despite the wide research carried on tissue regenerative approaches and biomaterials, only few products reached clinical market. ...
Another research path is using in vitro techniques for seed germination and determining the molecular mechanism of seedling development. This Special Issue will accept reviews and full or short research papers from a broad scope of interdisciplinary research on in vitro plant cultures, ranging from a single cell model to tissue cultures and ...
In contrast to other paper grades, tissue paper is often in intensive contact with the human skin, especially facial and toilet tissue. Besides the functionality, the subjective perception of the overall quality and the softness of the tissue plays a major role when it comes to a purchase decision of the costumer. de Assis et al. carried out comprehensive work on the importance of softness and ...
The resulting tissue paper will be added with additives that have antimicrobial properties of chitosan and mangosteen peel for the purpose of increasing the tensile strength or absorption of water. The aim of this research is to study the effect of depending variables (temperature and NaOH concentration) on chemical composition (cellulose and ...
Tissue paper is a lightweight paper or, light crêpe paper. Tissue can be made both from virgin and recycled paper pulp. Tissue is produced on a paper machine ... In 1951, William E. Corbin, Henry Chase (scientist), and Harold Titus began experimenting with paper towels in the Research and Development building of the Brown Company in Berlin ...
The life cycle of tissue paper manufactured. from virgin pulp includes the following processes: the wood logging, the pulp and chemicals making. processes, the tissue pap er production, and ...
Tissue engineering scaffolds are designed to influence the physical, chemical and biological environment surrounding a cell population. In this review we focus on our own work and introduce a range of strategies and materials used for tissue engineering, including the sources of cells suitable for tissue engineering: embryonic stem cells, bone marrow-derived mesenchymal stem cells and cord ...
ThesisPDF Available. Perceived Vs Recorded Quality of Tissue Paper: A Thematic Analysis of Online Customer Reviews. September 2019. DOI: 10.13140/RG.2.2.11935.43686. Thesis for: Master of Science ...
Adipose tissues are dynamic tissues that play crucial physiological roles in maintaining health and homeostasis. Although white adipose tissue and brown adipose tissue are currently considered key endocrine organs, they differ functionally and morphologically. The existence of the beige or brite adipocytes, cells displaying intermediary characteristics between white and brown adipocytes ...
The tissue paper market is currently experiencing rapid growth, owing to the high market demand, low carbon footprint, cost-effectiveness and accessibility, sustainability, as well as the distinctive properties of tissue products (Das et al. 2021; An et al. 2022).Tissue products have become an essential part in our daily life because of their unique advantages and benefits (Agarwal et al. 2023 ...
Additional markers of alterations in nerve tissue repair (SPON-1 and NFASC) were elevated in those with cognitive impairment and SCG3, suggestive of brain-gut axis disturbance, was elevated in ...
Promoting periodontal tissue regeneration and repairing periodontal bone defects is the ultimate treatment goal for periodontal disease, but the means and methods are very limited. Hydrogels are a class of highly hydrophilic polymer networks, and their good biocompatibility has made them a popular research material in the field of oral medicine ...
1. Introduction. Since the seminal paper of Langer and Vacanti over 25 years ago [], tissue engineering (TE) has grown enormously as a science and as an industry.In 1993, it was introduced to the wider scientific community as "an interdisciplinary field that applies the principles of engineering and the life sciences toward the development of biological substitutes that restore, maintain, or ...
Team publishes paper on tissue deformation tracking. ... Cristian Linte, associate professor; Richard Simon, research scientist; Zixin Yang; and Kelly Merrell, from the Chester F. Carlson Center for Imaging Science and Department of Biomedical Engineering, published "Boundary Constraint-free Biomechanical Model-Based Surface Matching for ...
The data presented in this article are related to the original research paper entitled "Comparative characterization of eucalyptus fibres and softwood fibres for tissue papers applications" available in Materials Letter: X Journal [1]. ... The handsheets produced were tested for various tissue paper properties like, thickness and bulk (ISO ...
However, research focused on gene-to-protein translation is also needed. Because of the limited availability of human lung tissue (especially in children) and with animal models of lung fibrosis recapitulating only partially the complexity of human disease, lung "organoids" of varying cellular components have recently emerged as novel ...