The Sapir-Whorf hypothesis and inference under uncertainty
Affiliation.
- 1 Department of Linguistics, Cognitive Science Program, University of California, Berkeley, CA.
- PMID: 29658198
- DOI: 10.1002/wcs.1464
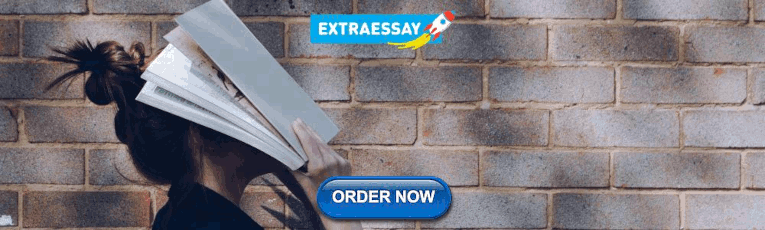
Publication types
- Published Erratum
UC Berkeley
The Sapir‐Whorf hypothesis and inference under uncertainty
- Regier, Terry ;
Published Web Location
On page 5, the symbol used in Equation 4 of Box 2 was incorrect; it should be “proportional to” (∝) instead of “equal” (=). The correct presentation of Equations 1–5 is as follows: (Formula presented.) (Formula presented.) (Formula presented.) (Formula presented.) (Formula presented.).
Many UC-authored scholarly publications are freely available on this site because of the UC's open access policies . Let us know how this access is important for you.
Europe PMC requires Javascript to function effectively.
Either your web browser doesn't support Javascript or it is currently turned off. In the latter case, please turn on Javascript support in your web browser and reload this page.
Search life-sciences literature (43,973,697 articles, preprints and more)
- Full text links
- Citations & impact
- Similar Articles
The Sapir-Whorf hypothesis and inference under uncertainty.
Author information, affiliations.
Wiley Interdisciplinary reviews. Cognitive Science , 28 Apr 2017 , 8(6) https://doi.org/10.1002/wcs.1440 PMID: 28455908
Abstract
Full text links .
Read article at publisher's site: https://doi.org/10.1002/wcs.1440
References
Articles referenced by this article (55)
The status of linguistics as a science
Language 1929
Title not supplied
, vii- 2012
AUTHOR UNKNOWN
Linguistic relativity.
Wolff P , Holmes KJ
Wiley Interdiscip Rev Cogn Sci, (3):253-265 2010
MED: 26302074
A study in language and cognition
J Abnorm Soc Psychol 1954
Citations & impact
Impact metrics, citations of article over time, alternative metrics.

Smart citations by scite.ai Smart citations by scite.ai include citation statements extracted from the full text of the citing article. The number of the statements may be higher than the number of citations provided by EuropePMC if one paper cites another multiple times or lower if scite has not yet processed some of the citing articles. Explore citation contexts and check if this article has been supported or disputed. https://scite.ai/reports/10.1002/wcs.1440
Article citations, written language acquisition is both shaped by and has an impact on brain functioning and cognition..
Front Hum Neurosci , 16:819956, 10 Jun 2022
Cited by: 0 articles | PMID: 35754773 | PMCID: PMC9226919
Semantic Expectation Effects on Object Detection: Using Figure Assignment to Elucidate Mechanisms.
Skocypec RM , Peterson MA
Vision (Basel) , 6(1):19, 21 Mar 2022
Cited by: 0 articles | PMID: 35324604 | PMCID: PMC8953613
Limits of a Second Language: Native and Second Languages in Management Team Communication.
Arnulf JK , Dai W , Lu H , Niu Z
Front Psychol , 12:580946, 21 Sep 2021
Cited by: 0 articles | PMID: 34621200 | PMCID: PMC8490803
Tracking within-category colors is easier: Color categories modulate location processing in a dynamic visual task.
Sun M , Hu L , Fan L , Zhang X
Mem Cognit , 48(1):32-41, 01 Jan 2020
Cited by: 0 articles | PMID: 31278633
No matter how: Top-down effects of verbal and semantic category knowledge on early visual perception.
Maier M , Abdel Rahman R
Cogn Affect Behav Neurosci , 19(4):859-876, 01 Aug 2019
Cited by: 3 articles | PMID: 30607831
Similar Articles
To arrive at the top five similar articles we use a word-weighted algorithm to compare words from the Title and Abstract of each citation.
The Sapir-Whorf Hypothesis and Probabilistic Inference: Evidence from the Domain of Color.
Cibelli E , Xu Y , Austerweil JL , Griffiths TL , Regier T
PLoS One , 11(7):e0158725, 19 Jul 2016
Cited by: 11 articles | PMID: 27434643 | PMCID: PMC4951127
[Rethinking the Sapir-Whorf hypothesis: role of language in shaping thought].
Shinrigaku Kenkyu , 71(5):415-433, 01 Dec 2000
Cited by: 0 articles | PMID: 11247288
Language, thought, and color: Whorf was half right.
Regier T , Kay P
Trends Cogn Sci , 13(10):439-446, 27 Aug 2009
Cited by: 67 articles | PMID: 19716754
Wiley Interdiscip Rev Cogn Sci , 2(3):253-265, 27 Oct 2010
Cited by: 35 articles | PMID: 26302074
Interactions between language, thought, and perception: Cognitive and neural perspectives.
Mahon BZ , Kemmerer D
Cogn Neuropsychol , 37(5-6):235-240, 01 Jul 2020
Cited by: 4 articles | PMID: 33172363
Funding
Funders who supported this work.
National Science Foundation (1)
Grant ID: SBE‐1041707
10 publication s
Europe PMC is part of the ELIXIR infrastructure

An official website of the United States government
The .gov means it’s official. Federal government websites often end in .gov or .mil. Before sharing sensitive information, make sure you’re on a federal government site.
The site is secure. The https:// ensures that you are connecting to the official website and that any information you provide is encrypted and transmitted securely.
- Publications
- Account settings
Preview improvements coming to the PMC website in October 2024. Learn More or Try it out now .
- Advanced Search
- Journal List

The Sapir-Whorf Hypothesis and Probabilistic Inference: Evidence from the Domain of Color
Emily cibelli.
1 Department of Linguistics, Northwestern University, Evanston, IL 60208, United States of America
2 Department of Linguistics, University of California, Berkeley, CA 94720, United States of America
3 Cognitive Science Program, University of California, Berkeley, CA 94720, United States of America
Joseph L. Austerweil
4 Department of Psychology, University of Wisconsin, Madison, WI 53706, United States of America
Thomas L. Griffiths
5 Department of Psychology, University of California, Berkeley, CA 94720, United States of America
Terry Regier
Conceived and designed the experiments: EC YX JLA TLG TR. Performed the experiments: EC YX JLA. Analyzed the data: EC YX JLA. Wrote the paper: TR EC YX.
Associated Data
All relevant data are available within the paper and/or at: https://github.com/yangxuch/probwhorfcolor This GitHub repository is mentioned in the paper.
The Sapir-Whorf hypothesis holds that our thoughts are shaped by our native language, and that speakers of different languages therefore think differently. This hypothesis is controversial in part because it appears to deny the possibility of a universal groundwork for human cognition, and in part because some findings taken to support it have not reliably replicated. We argue that considering this hypothesis through the lens of probabilistic inference has the potential to resolve both issues, at least with respect to certain prominent findings in the domain of color cognition. We explore a probabilistic model that is grounded in a presumed universal perceptual color space and in language-specific categories over that space. The model predicts that categories will most clearly affect color memory when perceptual information is uncertain. In line with earlier studies, we show that this model accounts for language-consistent biases in color reconstruction from memory in English speakers, modulated by uncertainty. We also show, to our knowledge for the first time, that such a model accounts for influential existing data on cross-language differences in color discrimination from memory, both within and across categories. We suggest that these ideas may help to clarify the debate over the Sapir-Whorf hypothesis.
Introduction
The Sapir-Whorf hypothesis [ 1 , 2 ] holds that our thoughts are shaped by our native language, and that speakers of different languages therefore think about the world in different ways. This proposal has been controversial for at least two reasons, both of which are well-exemplified in the semantic domain of color. The first source of controversy is that the hypothesis appears to undercut any possibility of a universal foundation for human cognition. This idea sits uneasily with the finding that variation in color naming across languages is constrained, such that certain patterns of color naming recur frequently across languages [ 3 – 5 ], suggesting some sort of underlying universal basis. The second source of controversy is that while some findings support the hypothesis, they do not always replicate reliably. Many studies have found that speakers of a given language remember and process color in a manner that reflects the color categories of their language [ 6 – 13 ]. Reinforcing the idea that language is implicated in these findings, it has been shown that the apparent effect of language on color cognition disappears when participants are given a verbal [ 7 ] (but not a visual) interference task [ 8 , 11 , 12 ]; this suggests that language may operate through on-line use of verbal representations that can be temporarily disabled. However, some of these findings have a mixed record of replication [ 14 – 17 ]. Thus, despite the substantial empirical evidence already available, the role of language in color cognition remains disputed.
An existing theoretical stance holds the potential to resolve both sources of controversy. On the one hand, it explains effects of language on cognition in a framework that retains a universal component, building on a proposal by Kay and Kempton [ 7 ]. On the other hand, it has the potential to explain when effects of language on color cognition will appear, and when they will not—and why. This existing stance is that of the “category adjustment” model of Huttenlocher and colleagues [ 18 , 19 ]. We adopt this stance, and cast color memory as inference under uncertainty, instantiated in a category adjustment model, following Bae et al. [ 20 ] and Persaud and Hemmer [ 21 ]. The model holds that color memory involves the probabilistic combination of evidence from two sources: a fine-grained representation of the particular color seen, and the language-specific category in which it fell (e.g. English green ). Both sources of evidence are represented in a universal perceptual color space, yet their combination yields language-specific bias patterns in memory, as illustrated in Fig 1 . The model predicts that such category effects will be strongest when fine-grained perceptual information is uncertain. It thus has the potential to explain the mixed pattern of replications of Whorfian effects in the literature: non-replications could be the result of high perceptual certainty.

A stimulus is encoded in two ways: (1) a fine-grained representation of the stimulus itself, shown as a (gray) distribution over stimulus space centered at the stimulus’ location in that space, and (2) the language-specific category (e.g. English “green”) in which the stimulus falls, shown as a separate (green) distribution over the same space, centered at the category prototype. The stimulus is reconstructed by combining these two sources of information through probabilistic inference, resulting in a reconstruction of the stimulus (black distribution) that is biased toward the category prototype. Adapted from Fig 11 of Bae et al. (2015) [ 20 ].
In the category adjustment model, both the fine-grained representation of the stimulus and the category in which it falls are modeled as probability distributions over a universal perceptual color space. The fine-grained representation is veridical (unbiased) but inexact: its distribution is centered at the location in color space where the stimulus itself fell, and the variance of that distribution captures the observer’s uncertainty about the precise location of the stimulus in color space, with greater variance corresponding to greater uncertainty. Psychologically, such uncertainty might be caused by noise in perception itself, by memory decay over time, or by some other cause—and any increase in such uncertainty is modeled by a wider, flatter distribution for the fine-grained representation. The category distribution, in contrast, captures the information about stimulus location that is given by the named category in which the stimulus fell (e.g. green for an English-speaking observer). Because named color categories vary across languages, this category distribution is assumed to be language-specific—although the space over which it exists is universal. The model infers the original stimulus location by combining evidence from both of these distributions. As a result, the model tends to produce reconstructions of the stimulus that are biased away from the actual location of the stimulus and toward the prototype of the category in which it falls.
As illustrated in Fig 2 , this pattern of bias pulls stimuli on opposite sides of a category boundary in opposite directions, producing enhanced distinctiveness for such stimuli. Such enhanced distinctiveness across a category boundary is the signature of categorical perception, or analogous category effects in memory. On this view, language-specific effects on memory can emerge from a largely universal substrate when one critical component of that substrate is language-specific: the category distribution.

Model reconstructions tend to be biased toward category prototypes, yielding enhanced distinctiveness for two stimuli that fall on different sides of a category boundary. Categories are shown as distributions in green and blue; stimuli are shown as vertical black lines; reconstruction bias patterns are shown as arrows.
If supported, the category adjustment model holds the potential to clarify the debate over the Sapir-Whorf hypothesis in three ways. First, it would link that debate to independent principles of probabilistic inference. In so doing, it would underscore the potentially important role of uncertainty , whether originating in memory or perception, in framing the debate theoretically. Second, and relatedly, it would suggest a possible reason why effects of language on color memory and perception are sometimes found, and sometimes not [ 17 ]. Concretely, the model predicts that greater uncertainty in the fine-grained representation—induced for example through a memory delay, or noise in perception—will lead to greater influence of the category, and thus a stronger bias in reproduction. The mirror-image of this prediction is that in situations of relatively high certainty in memory or perception, there will be little influence of the category, to the point that such an influence may not be empirically detectable. Third, the model suggests a way to think about the Sapir-Whorf hypothesis without jettisoning the important idea of a universal foundation for cognition.
Closely related ideas appear in the literature on probabilistic cue integration [ 22 – 25 ]. For example, Ernst and Banks [ 24 ] investigated perceptual integration of cues from vision and touch in judging the height of an object. They found that humans integrate visual and haptic cues in a statistically optimal fashion, modulated by cue certainty. The category adjustment model we explore here can be seen as a form of probabilistic cue integration in which one of the cues is a language-specific category.
The category adjustment model has been used to account for category effects in various domains, including spatial location [ 18 , 26 ], object size [ 19 , 27 ], and vowel perception [ 28 ]. The category adjustment model also bears similarities to other theoretical accounts of the Sapir-Whorf hypothesis that emphasize the importance of verbal codes [ 7 , 8 ], and the interplay of such codes with perceptual representations [ 29 – 31 ]. Prior research has linked such category effects to probabilistic inference, following the work of Huttenlocher and colleagues [ 18 , 19 ]. Roberson and colleagues [ 32 ] invoked the category adjustment model as a possible explanation for categorical perception of facial expressions, but did not explore a formal computational model; Goldstone [ 33 ] similarly referenced the category adjustment model with respect to category effects in the color domain. Persaud and Hemmer [ 21 , 34 ] explored bias in memory for color, and compared empirically obtained memory bias patterns from English speakers with results predicted by a formally specified category adjustment model, but did not link those results to the debate over the Sapir-Whorf hypothesis, and did not manipulate uncertainty. More recently, a subsequent paper by the same authors and colleagues [ 35 ] explored category-induced bias in speakers of another language, Tsimané, and did situate those results with respect to the Sapir-Whorf hypothesis, but again did not manipulate uncertainty. Most recently, Bae et al. [ 20 ] extensively documented bias in color memory in English speakers, modeled those results with a category-adjustment computational model, and did manipulate uncertainty—but did not explore these ideas relative to the Sapir-Whorf hypothesis, or to data from different languages.
In what follows, we first present data and computational simulations that support the recent finding that color memory in English speakers is well-predicted by a category adjustment model, with the strength of category effects modulated by uncertainty. We then show, to our knowledge for the first time, that a category adjustment model accounts for influential existing cross-language data on color that support the Sapir-Whorf hypothesis.
In this section we provide general descriptions of our analyses and results. Full details are supplied in the section on Materials and Methods.
Study 1: Color reconstruction in English speakers
Our first study tests the core assumptions of the category adjustment model in English speakers. In doing so, it probes questions that were pursued by two studies that appeared recently, after this work had begun. Persaud and Hemmer [ 21 ] and Bae et al. [ 20 ] both showed that English speakers’ memory for a color tends to be biased toward the category prototype of the corresponding English color term, in line with a category adjustment model. Bae et al. [ 20 ] also showed that the amount of such bias increases when subjects must retain the stimulus in memory during a delay period, compared to when there is no such delay, as predicted by the principles of the category adjustment model. In our first study, we consider new evidence from English speakers that tests these questions, prior to considering speakers of different languages in our following studies.
English-speaking participants viewed a set of hues that varied in small steps from dark yellow to purple, with most hues corresponding to some variety of either green or blue. We collected two kinds of data from these participants: bias data and naming data. Bias data were based on participants’ non-linguistic reconstruction of particular colors seen. Specifically, for each hue seen, participants recreated that hue by selecting a color from a color wheel, either while the target was still visible ( Fig 3A : simultaneous condition), or from memory after a short delay ( Fig 3B : delayed condition). We refer to the resulting data as bias data, because we are interested in the extent to which participants’ reconstructions of the stimulus color are biased away from the original target stimulus. Afterwards, the same participants indicated how good an example of English green (as in Fig 3C ) and how good an example of English blue each hue was. We refer to these linguistic data as naming data.

Screenshots of example trials illustrating (A) simultaneous reconstruction, (B) delayed reconstruction, and (C) green goodness rating.
Fig 4 shows both naming and bias data as a function of target hue. The top panel of the figure shows the naming data and also shows Gaussian functions corresponding to the English color terms green and blue that we fitted to the naming data. Bias data were collected for only a subset of the hues for which naming data were collected, and the shaded region in the top panel of Fig 4 shows that subset, relative to the full range of hues for naming data. We collected bias data only in this smaller range because we were interested specifically in bias induced by the two color terms blue and green , and colors outside the shaded region seemed to us to clearly show some influence of neighboring categories such as yellow and purple . The bottom panel of the figure shows the bias data, plotted relative to the prototypes (means) of the fitted Gaussian functions for green and blue . It can be seen that reconstruction bias appears to be stronger in the delayed than in the simultaneous condition, as predicted, and that—especially in the delayed condition—there is an inflection in the bias pattern between the two category prototypes, suggesting that bias may reflect the influence of each of the two categories. The smaller shaded region in this bottom panel denotes the subset of these hues that we subsequently analyzed statistically, and to which we fit models. We reduced the range of considered hues slightly further at this stage, to ensure that the range was well-centered with respect to the two relevant category prototypes, for green and blue , as determined by the naming data.

In both top and bottom panels, the horizontal axis denotes target hue, ranging from yellow on the left to purple on the right. Top panel (naming data): The solid green and blue curves show, for each target hue, the average goodness rating for English green and blue respectively, as a proportion of the maximum rating possible. The dashed green and blue curves show Gaussian functions fitted to the naming goodness data. The dotted vertical lines marked at the bottom with green and blue squares denote the prototypes for green and blue , determined as the means of the green and blue fitted Gaussian functions, respectively. The shaded region in the top panel shows the portion of the spectrum for which bias data were collected. Bottom panel (bias data): Solid curves denote, for each target hue, the average reconstruction bias for that hue, such that positive values denote reconstruction bias toward the purple (here, right) end of the spectrum, and negative values denote reconstruction bias toward the yellow (here, left) end of the spectrum. Units for the vertical axis are the same as for the horizontal axis, which is normalized to length 1.0. The black and red curves show bias under simultaneous and delayed response, respectively. Blue stars at the top of the bottom panel mark hues for which there was a significant difference in the magnitude of bias between simultaneous and delayed conditions. The shaded region in the bottom panel shows the portion of the data that was analyzed statistically, and to which models were fit. In both panels, error bars represent standard error of the mean.
The absolute values (magnitudes) of the bias were analyzed using a 2 (condition: simultaneous vs. delayed) × 15 (hues) repeated measures analysis of variance. This analysis revealed significantly greater bias magnitude in the delayed than in the simultaneous condition. It also revealed that bias magnitude differed significantly as a function of hue, as well as a significant interaction between the factors of hue and condition. The blue stars in Fig 4 denote hues for which the difference in bias magnitude between the simultaneous and delayed conditions reached significance. The finding of greater bias magnitude in the delayed than in the simultaneous condition is consistent with the proposal that uncertainty is an important mediating factor in such category effects, as argued by Bae et al. [ 20 ]. It also suggests that some documented failures to find such category effects could in principle be attributable to high certainty, a possibility that can be explored by manipulating uncertainty.
We wished to test in a more targeted fashion to what extent these data are consistent with a category adjustment model in which a color is reconstructed based in part on English named color categories. To that end, we compared the performance of four models against these data; only one of these models considered both of the relevant English color categories, green and blue . As in Fig 1 , each model contains a fine-grained but inexact representation of the perceived stimulus, and (for most models) a representation of one or more English color categories. Each model predicts the reconstruction of the target stimulus from its fine-grained representation of the target together with any category information. Category information in the model is specified by the naming data. Each model has a single free parameter, corresponding to the uncertainty of the fine-grained representation; this parameter is fit to bias data.
- The null model is a baseline model that predicts hue reconstruction based only on the fine-grained representation of the stimulus, with no category component.
- The 1-category (green) model predicts hue reconstruction based on the fine-grained representation of the stimulus, combined with a representation of only the green category, derived from the green naming data.
- The 1-category (blue) model predicts hue reconstruction based on the fine-grained representation of the stimulus, combined with a representation of only the blue category, derived from the blue naming data.
- The 2-category model predicts hue reconstruction based on the fine-grained representation of the stimulus, combined with representations of both the green and blue categories.
If reproduction bias reflects probabilistic inference from a fine-grained representation of the stimulus itself, together with any relevant category, we would expect the 2-category model to outperform the others. The other models have access either to no category information at all (null model), or to category information for only one of the two relevant color categories (only one of green and blue ). The 2-category model in contrast combines fine-grained stimulus information with both of the relevant categories ( green and blue ); this model thus corresponds most closely to a full category adjustment model.
Fig 5 redisplays the data from simultaneous and delayed reconstruction, this time with model fits overlaid. The panels in the left column show data from simultaneous reconstruction, fit by each of the four models, and the panels in the right column analogously show data and model fits from delayed reconstruction. Visually, it appears that in the case of delayed reconstruction, the 2-category model fits the data at least qualitatively better than competing models: it shows an inflection in bias as the empirical data do, although not as strongly. For simultaneous reconstruction, the 2-category model fit is also reasonable but visually not as clearly superior to the others (especially the null model) as in the delayed condition.

Left column: Bias from simultaneous reconstruction, fit by each of the four models. The empirical data (black lines with error bars) in these four panels are the same, and only the model fits (red lines) differ. Within each panel, the horizontal axis denotes target hue, and the vertical axis denotes reconstruction bias. The green and blue prototypes are indicated as vertical lines with green and blue squares at the bottom. Right column: delayed reconstruction, displayed analogously.
Table 1 reports quantitative results of these model fits. The best fit is provided by the 2-category model, in both the simultaneous and delayed conditions, whether assessed by log likelihood (LL) or by mean squared errror (MSE). In line with earlier studies [ 20 , 21 ], these findings demonstrate that a category adjustment model that assumes stimulus reconstruction is governed by relevant English color terms provides a reasonable fit to data on color reconstruction by English speakers. The category adjustment model fits well both when the category bias is relatively slight (simultaneous condition), and when the bias is stronger (delayed condition).
LL = log likelihood (higher is better). MSE = mean squared error (lower is better). The best value in each row is shown in bold .
Study 2: Color discrimination across languages
The study above examined the categories of just one language, English, whereas the Sapir-Whorf hypothesis concerns cross-language differences in categorization, and their effect on cognition and perception. Empirical work concerning this hypothesis has not specifically emphasized bias in reconstruction, but there is a substantial amount of cross-language data of other sorts against which the category adjustment model can be assessed. One method that has been extensively used to explore the Sapir-Whorf hypothesis in the domain of color is a two-alternative forced choice (2AFC) task. In such a task, participants first are briefly shown a target color, and then shortly afterward are shown that same target color together with a different distractor color, and are asked to indicate which was the color originally seen. A general finding from such studies [ 8 – 10 ] is that participants exhibit enhanced discrimination for pairs of colors that would be named differently in their native language. For example, in such a 2AFC task, speakers of English show enhanced discrimination for colors from the different English categories green and blue , compared with colors from the same category (either both green or both blue ) [ 8 ]. In contrast, speakers of the Berinmo language, which has named color categories that differ from those of English, show enhanced discrimination across Berinmo category boundaries, and not across those of English [ 9 ]. Thus color discrimination in this task is enhanced at the boundaries of native language categories, suggesting an effect of those native language categories on the ability to discriminate colors from memory.
Considered informally, this qualitative pattern of results appears to be consistent with the category adjustment model, as suggested above in Fig 2 . We wished to determine whether such a model would also provide a good quantitative account of such results, when assessed using the specific color stimuli and native-language naming patterns considered in the empirical studies just referenced.
We considered cross-language results from two previous studies by Debi Roberson and colleagues, one that compared color memory in speakers of English and Berinmo, a language of Papua New Guinea [ 9 ], and another that explored color memory in speakers of Himba, a language of Namibia [ 10 ]. Berinmo and Himba each have five basic color terms, in contrast with eleven in English. The Berinmo and Himba color category systems are similar to each other in broad outline, but nonetheless differ noticeably. Following these two previous studies, we considered the following pairs of categories in these three languages:
- the English categories green and blue ,
- the Berinmo categories wor (covering roughly yellow, orange, and brown), and nol (covering roughly green, blue, and purple), and
- the Himba categories dumbu (covering roughly yellow and beige) and burou (covering roughly green, blue, and purple).
These three pairs of categories are illustrated in Fig 6 , using naming data from Roberson et al. (2000) [ 9 ] and Roberson et al. (2005) [ 10 ]. It can be seen that the English green - blue distinction is quite different from the Berinmo wor - nol and the Himba dumbu - burou distinctions, which are similar but not identical to each other. The shaded regions in this figure indicate specific colors that were probed in discrimination tasks. The shaded (probed) region that straddles a category boundary in Berinmo and Himba falls entirely within the English category green , and the shaded (probed) region that straddles a category boundary in English falls entirely within the Berinmo category nol and the Himba category burou , according to naming data in Fig 1 of Roberson et al. (2005) [ 10 ]. The empirical discrimination data in Fig 7 are based on those probed colors [ 9 , 10 ], and show that in general, speakers of a language tend to exhibit greater discrimination for pairs of colors that cross a category boundary in their native language, consistent with the Sapir-Whorf hypothesis.

The English categories green and blue (top panel), the Berinmo categories wor and nol (middle panel), and the Himba categories dumbu and burou (bottom panel), plotted against a spectrum of hues that ranges from dark yellow at the left, through green, to blue at the right. Colored squares mark prototypes: the shared prototype for Berinmo wor and Himba dumbu , and the prototypes for English green and blue ; the color of each square approximates the color of the corresponding prototype. For each language, the dotted-and-dashed vertical lines denote the prototypes for the two categories from that language, and the dashed vertical line denotes the empirical boundary between these two categories. Black curves show the probability of assigning a given hue to each of the two native-language categories, according to the category component of a 2-category model fit to each language’s naming data. The shaded regions mark the ranges of colors probed in discrimination tasks; these two regions are centered at the English green - blue boundary and the Berinmo wor - nol boundary. Data are from Roberson et al. (2000) [ 9 ] and Roberson et al. (2005) [ 10 ].

Top panels: Discrimination from memory by Berinmo and English speakers for pairs of colors across and within English and Berinmo color category boundaries. Empirical data are from Table 11 of Roberson et al. (2000:392). Empirical values show mean proportion correct 2AFC memory judgments, and error bars show standard error. Model values show mean model proportion correct 2AFC memory judgments after simulated reconstruction with native-language categories. Model results are range-matched to the corresponding empirical values, such that the minimum and maximum model values match the minimum and maximum mean values in the corresponding empirical dataset, and other model values are linearly interpolated. Bottom panels: Discrimination from memory by Himba and English speakers for pairs of colors across and within English and Himba color category boundaries, compared with model results based on native-language categories. Empirical data are from Table 6 of Roberson et al. (2005:400); no error bars are shown because standard error was not reported in that table.
We sought to determine whether the 2-category model explored above could account for these data. To that end, for each language, we created a version of the 2-category model based on the naming data for that language. Thus, we created an English model in which the two categories were based on empirical naming data for green and blue , a Berinmo model in which the two categories were based on empirical naming data for wor and nol , and a Himba model in which the two categories were based on empirical naming data for dumbu and burou . The black curves in Fig 6 show the probability of assigning a given hue to each of the two native-language categories, according to the category component of a 2-category model fit to each language’s naming data. Given this category information, we simulated color reconstruction from memory for the specific colors considered in the empirical studies [ 9 , 10 ] (the colors in the shaded regions in Fig 6 ). We did so separately for the cases of English, Berinmo, and Himba, in each case fitting a model based on naming data for a given language to discrimination data from speakers of that language. As in Study 1, we fit the model parameter corresponding to the uncertainty of fine-grained perceptual representation to the empirical non-linguistic (here discrimination) data, and we used a single value for this parameter across all three language models. The model results are shown in Fig 7 , beside the empirical data to which they were fit. The models provide a reasonable match to the observed cross-language differences in discrimination. Specifically, the stimulus pairs for which empirical performance is best are those that cross a native-language boundary—and these are stimulus pairs for which the corresponding model response is strongest.
Although not shown in the figure, we also conducted a followup analysis to test whether the quality of these fits was attributable merely to model flexibility, or to a genuine fit between a language’s category system and patterns of discrimination from speakers of that language. We did this by switching which language’s model was fit to which language’s discrimination data. Specifically, we fit the model based on Berinmo naming to the discrimination data from English speakers (and vice versa), and fit the model based on Himba naming to the discrimination data from English speakers (and vice versa), again adjusting the model parameter corresponding to the uncertainty of the fine-grained perceptual representation to the empirical discrimination data. The results are summarized in Table 2 . It can be seen that the discrimination data are fit better by native-language models (that is, models with a category component originally fit to that language’s naming data) than by other-language models (that is, models with a category component originally fit to another language’s naming data). These results suggest that cross-language differences in discrimination may result from category-induced reconstruction bias under uncertainty, guided by native-language categories.
The best value in each row is shown in bold . Data are fit better by native-language models than by other-language models.
Study 3: Within-category effects
Although many studies of categorical perception focus on pairs of stimuli that cross category boundaries, there is also evidence for category effects within categories. In a 2AFC study of categorical perception of facial expressions, Roberson and colleagues [ 32 ] found the behavioral signature of categorical perception (or more precisely in this case, categorical memory): superior discrimination for cross-category than for within-category pairs of stimuli. But in addition, they found an interesting category effect on within-category pairs, dependent on order of presentation. For each within-category pair they considered, one stimulus of the pair was always closer to the category prototype (the “good exemplar”) than the other (the “poor exemplar”). They found that 2AFC performance on within-category pairs was better when the target was the good exemplar (and the distractor was therefore the poor exemplar) than when the target was the poor exemplar (and the distractor was therefore the good exemplar)—even though the same stimuli were involved in the two cases. Moreover, performance in the former (good exemplar) case did not differ significantly from cross-category performance. Hanley and Roberson [ 36 ] subsequently reanalyzed data from a number of earlier studies that had used 2AFC tasks to explore cross-language differences in color naming and cognition, including those reviewed and modeled in the previous section. Across studies and across domains, including color, they found the same asymmetrical within-category effect originally documented for facial expressions.
This within-category pattern may be naturally explained in category-adjustment terms, as shown in Fig 8 , and as argued by Roberson and colleagues [ 32 ]. The central idea is that because the target is held in memory, it is subject to bias toward the prototype in memory, making discrimination of target from distractor either easier or harder depending on which of the two stimuli is the target. Although this connection with the category adjustment model has been made in the literature in general conceptual terms [ 32 ], followup studies have been theoretically focused elsewhere [ 31 , 36 ], and the idea has not to our knowledge been tested computationally using the specific stimuli and naming patterns involved in the empirical studies. We sought to do so.

The category adjustment model predicts: (top panel, good exemplar) easy within-category discrimination in a 2AFC task when the initially-presented target t is closer to the prototype than the distractor d is; (bottom panel, poor exemplar) difficult within-category discrimination with the same two stimuli when the initially-presented target t is farther from the prototype than the distractor d is. Category is shown as a distribution in blue; stimuli are shown as vertical black lines marked t and d; reconstruction bias patterns are shown as arrows.
The empirical data in Fig 9 illustrate the within-category effect with published results on color discrimination by speakers of English, Berinmo, and Himba. In attempting to account for these data, we considered again the English, Berinmo, and Himba variants of the 2-category model first used in Study 2, and also retained from that study the parameter value corresponding to the uncertainty of the fine-grained perceptual representation, in the case of native-language models. We simulated reconstruction from memory of the specific colors examined in Study 2. Following the empirical analyses, this time we disaggregated the within-category stimulus pairs into those in which the target was a good exemplar of the category (i.e. the target was closer to the prototype than the distractor was), vs. those in which the target was a poor exemplar of the category (i.e. the target was farther from the prototype than the distractor was). The model results are shown in Fig 9 , and match the empirical data reasonably well, supporting the informal in-principle argument of Fig 8 with a more detailed quantitative analysis.

Across: stimulus pair crosses the native-language boundary; GE: within-category pair, target is the good exemplar; PE: within-category pair, target is the poor exemplar. Empirical data are from Figs 2 (English: 10-second retention interval), 3 (Berinmo), and 4 (Himba) of Hanley and Roberson [ 36 ]. Empirical values show mean proportion correct 2AFC memory judgments, and error bars show standard error. Model values show mean model proportion correct 2AFC memory judgments after simulated reconstruction using native-language categories, range-matched as in Fig 7 . English model compared with English data: 0.00002 MSE; Berinmo model compared with Berinmo data: 0.00055 MSE; Himba model compared with Himba data: 0.00087 MSE.
Conclusions
We have argued that the debate over the Sapir-Whorf hypothesis may be clarified by viewing that hypothesis in terms of probabilistic inference. To that end, we have presented a probabilistic model of color memory, building on proposals in the literature. The model assumes both a universal color space and language-specific categorical partitionings of that space, and infers the originally perceived color from these two sources of evidence. The structure of this model maps naturally onto a prominent proposal in the literature that has to our knowledge not previously been formalized in these terms. In a classic early study of the effect of language on color cognition, Kay and Kempton [ 7 ] interpret Whorf [ 2 ] as follows:
Whorf […] suggests that he conceives of experience as having two tiers: one, a kind of rock bottom, inescapable seeing-things-as-they-are (or at least as human beings cannot help but see them), and a second, in which [the specific structures of a given language] cause us to classify things in ways that could be otherwise (and are otherwise for speakers of a different language).
Kay and Kempton argue that color cognition involves an interaction between these two tiers. The existence of a universal groundwork for color cognition helps to explain why there are constraints on color naming systems across languages [ 3 – 5 , 37 ]. At the same time, Kay and Kempton acknowledge a role for the language-specific tier in cognition, such that “there do appear to be incursions of linguistic categorization into apparently nonlinguistic processes of thinking” (p. 77). These two tiers map naturally onto the universal and language-specific components of the model we have explored here. This structure offers a straightforward way to think about effects of language on cognition while retaining the idea of a universal foundation underpinning human perception and cognition. Thus, this general approach, and our model as an instance of it, offer a possible resolution of one source of controversy surrounding the Sapir-Whorf hypothesis: taking that hypothesis seriously need not entail a wholesale rejection of important universal components of human cognition.
The approach proposed here also has the potential to resolve another source of controversy surrounding the Sapir-Whorf hypothesis: that some findings taken to support it do not replicate reliably (e.g. in the case of color: [ 15 – 17 ]). Framing the issue in terms of probabilistic inference touches this question by highlighting the theoretically central role of uncertainty , as in models of probabilistic cue integration [ 24 ]. We have seen stronger category-induced bias in color memory under conditions of greater delay and presumably therefore greater uncertainty (Study 1, and [ 20 ]). This suggests that in the inverse case of high certainty about the stimulus, any category effect could in principle be so small as to be empirically undetectable, a possibility that can be pursued by systematically manipulating uncertainty. Thus, the account advanced here casts the Sapir-Whorf hypothesis in formal terms that suggest targeted and quantitative followup tests. A related theoretical advantage of uncertainty is that it highlights an important level of generality: uncertainty could result from memory, as explored here, but it could also result from noise or ambiguity in perception itself, and on the view advanced here, the result should be the same.
The model we have proposed does not cover all aspects of language effects on color cognition. For example, there are documented priming effects [ 31 ] which do not appear to flow as naturally from this account as do the other effects we have explored above. However, the model does bring together disparate bodies of data in a simple framework, and links them to independent principles of probabilistic inference. Future research can usefully probe the generality and the limitations of the ideas we have explored here.
Materials and Methods
Code and data supporting the analyses reported here are available at https://github.com/yangxuch/probwhorfcolor.git .
The basic model we consider is shown in Fig 10 , which presents in graphical form the generative process behind Fig 1 above. Our model follows in general outline that of Bae et al. [ 20 ], but the formalization of inference within this structure more closely follows Feldman et al.’s [ 28 ] model of category effects in vowel perception. In our model, the perception of a stimulus S = s produces a fine-grained memory M , and a categorical code c . We wish to obtain a reconstruction s ^ of the original stimulus S = s , by combining evidence from the two internal representations M and c that s has produced. That reconstruction is derived as follows:
Because hue is a circular dimension, the components p ( M | S ) and p ( S | c ) could be modeled using circular normal or von Mises distributions, as was done by Bae et al. [ 20 ]. However each of our studies treats only a restricted subsection of the full hue circle, and for that reason we instead model these representations using normal distributions.

The perception of stimulus S = s produces a fine-grained memory M , and a categorical code c specifying the category in which s fell. We wish to reconstruct the original stimulus S = s , given M and c .
p ( M | S ) represents the fine-grained memory trace M of the original stimulus S = s . We model this as a normal distribution with mean μ m at the location of the original stimulus s , and with uncertainty captured by variance σ m 2 :
This is an unbiased representation of the original stimulus s because μ m = s .
p ( S | c ) captures the information about the location of stimulus S that is given by the categorical code c . We again model this as a normal distribution, this time centered at the prototype μ c of category c , with variance σ c 2 :
This assumes that there is a single categorical code c , and we use this assumption in some of our model variants below. However in other cases we will capture the fact that more than one category may be applicable to a stimulus. In such cases we assume that the perceiver knows, for each category c , the applicability π ( c ) of that category for the observed stimulus s . We model this as:
where p ( S = s | c ) is given by Eq (4) above, and p ( c ) is assumed to be uniform.
We consider three variants of this basic model, described below in order of increasing complexity: the null model, the 1-category model, and the 2-category model. For each model, we take the predicted reconstruction s ^ of a given stimulus S = s to be the expected value of the posterior distribution:
The null model assumes that reconstruction is based only on the fine-grained memory, with no category influence. This model is derived from Eq (2) by assuming that the memory component p ( M | S ) is as defined above, and the category component p ( S | c ) is uniform, yielding:
The predicted reconstruction for this model is given by the expected value of this distribution, namely:
where we have assumed μ m = s , the originally observed stimulus. This model predicts no category-induced bias: the reconstruction of the stimulus S = s is simply the value of the stimulus s itself.
1-category model
The 1-category model assumes that reconstruction is based both on fine-grained memory and on information from a single category, e.g. English green . This model is derived from Eq (2) by assuming that both the memory component p ( M | S ) and the category component p ( S | c ) are as defined above, yielding:
where we have assumed μ m = s , the originally observed stimulus. This equation parallels Eq (7) of Feldman et al. [ 28 ]. This model produces a reconstruction that is a weighted average of the original stimulus value s and the category prototype μ c , with weights determined by the relative certainty of each of the two sources of information. The same weighted average is also central to Ernst and Banks’ [ 24 ] study of cue integration from visual and haptic modalities. That study was based on the same principles we invoke here, and our model—like that of Feldman et al.—can be viewed as a probabilistic cue integration model in which one of the two cues being integrated is a category, rather than a cue from a different modality.
2-category model
The 2-category model is similar to the 1-category model, but instead of basing its reconstruction on a single category c , it bases its reconstruction on two categories c 1 and c 2 (e.g. English green and blue ). It does so by averaging together the reconstruction provided by the 1-category model for c 1 and the reconstruction provided by the 1-category model for c 2 , weighted by the applicability π ( c ) of each category c to the stimulus:
Here, p ( S | M , c ) inside the sum is given by the 1-category model specified in Eq (9) , and π ( c ) is the applicability of category c to the observed stimulus s as specified above in Eq (5) . Our equation here parallels Eq (9) of Feldman et al. [ 28 ] who similarly take a weighted average over 1-category models in their model of category effects in speech perception. The predicted reconstruction for this model is given by the expected value of this distribution, namely:
assuming as before that μ m = s , the original stimulus value. This equation follows Feldman et al. [ 28 ] Eq (10).
Fitting models to data
For each model, any category parameters μ c and σ c 2 are first fit to naming data. The single remaining free parameter σ m 2 , corresponding to the uncertainty of fine-grained memory, is then fit to non-linguistic bias or discrimination data, with no further adjustment of the category parameters. Although this two-step process is used in all of our studies, it is conducted in slightly different ways across studies; we supply study-specific details below in our presentation of each study. All model fits were done using fminsearch in Matlab.
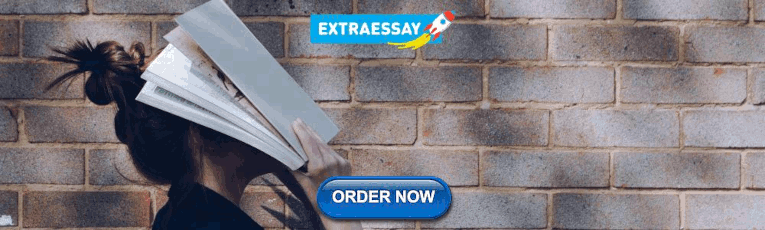
Participants
Twenty subjects participated in the experiment, having been recruited at UC Berkeley. All subjects were at least 18 years of age, native English speakers, and reported normal or corrected-to-normal vision, and no colorblindness. All subjects received payment or course credit for participation.
Informed consent was obtained verbally; all subjects read an approved consent form and verbally acknowledged their willingness to participate in the study. Verbal consent was chosen because the primary risk to subjects in this study was for their names to be associated with their response; this approach allowed us to obtain consent and collect data without the need to store subjects’ names in any form. Once subjects acknowledged that they understood the procedures and agreed to participate by stating so to the experimenter, the experimenter recorded their consent by assigning them a subject number, which was anonymously linked to their data. All study procedures, including those involving consent, were overseen and approved by the UC Berkeley Committee for the Protection of Human Subjects.
Stimuli were selected by varying a set of hues centered around the blue - green boundary, holding saturation and lightness constant. Stimuli were defined in Munsell coordinate space, which is widely used in the literature we engage here (e.g. [ 9 , 10 ]). All stimuli were at lightness 6 and saturation 8. Hue varied from 5Y to 10P, in equal hue steps of 2.5. Colors were converted to xyY coordinate space following Table I(6.6.1) of Wyszecki and Stiles (1982) [ 38 ]. The colors were implemented in Matlab in xyY; the correspondence of these coordinate systems in the stimulus set, as well as approximate visualizations of the stimuli, are reported in Table 3 .
All stimuli were presented at lightness 6, saturation 8 in Munsell space.
We considered three progressively narrower ranges of these stimuli for different aspects of our analyses, in an attempt to focus the analyses on a region that is well-centered relative to the English color categories green and blue . We refer to these three progressively narrower ranges as the full range , the medium range , and the focused range . We specify these ranges below, together with the aspects of the analysis for which each was used.
- Full range: We collected naming data for green and blue relative to the full range, stimuli 1-27, for a total of 27 stimuli. We fit the category components of our models to naming data over this full range.
- Medium range: We collected bias data for a subset of the full range, namely the medium range, stimuli 5-23, for a total of 19 stimuli. We considered this subset because we were interested in bias induced by the English color terms green and blue , and we had the impression, prior to collecting naming or bias data, that colors outside this medium range had some substantial element of the neighboring categories yellow and purple .
- Focused range: Once we had naming data, we narrowed the range further based on those data, to the focused range, stimuli 5-19, for a total of 15 stimuli. The focused range extends between the (now empirically assessed) prototypes for green and blue , and also includes three of our stimulus hues on either side of these prototypes, yielding a range well-centered relative to those prototypes, as can be seen in the bottom panel of Fig 4 above. We considered this range in our statistical analyses, and in our modeling of bias patterns.
Experimental procedure
The experiment consisted of four blocks. The first two blocks were reconstruction (bias) tasks: one simultaneous block and one delay block. In the simultaneous block ( Fig 3A ), the subject was shown a stimulus color as a colored square (labeled as “Original” in the figure), and was asked to recreate that color in a second colored square (labeled as “Target” in the figure) as accurately as possible by selecting a hue from a color wheel. The (“Original”) stimulus color remained on screen while the subject selected a response from the color wheel; navigation of the color wheel would change the color of the response (“Target”) square. The stimulus square and response square each covered 4.5 degrees of visual angle, and the color wheel covered 11.1 degrees of visual angle. Target colors were drawn from the medium range of stimuli (stimuli 5—23 of Table 3 ). The color wheel was constructed based on the full range of stimuli (stimuli 1—27 of Table 3 ), supplemented by interpolating 25 points evenly in xyY coordinates between each neighboring pair of the 27 stimuli of the full range, to create a finely discretized continuum from yellow to purple, with 677 possible responses. Each of the 19 target colors of the medium range was presented five times per block in random order, for a total of 95 trials per block. The delay block ( Fig 3B ) was similar to the simultaneous block but with the difference that the stimulus color was shown for 500 milliseconds then disappeared, then a fixation cross was shown for 1000 milliseconds, after which the subject was asked to reconstruct the target color from memory, again using the color wheel to change the color of the response square. The one colored square shown in the final frame of Fig 3B is the response square that changed color under participant control. The order of the simultaneous block and delay block were counterbalanced by subject. Trials were presented with a 500 millisecond inter-trial interval.
Several steps were taken to ensure that responses made on the color wheel during the reconstruction blocks were not influenced by bias towards a particular spatial position. The position of the color wheel was randomly rotated up to 180 degrees from trial to trial. The starting position of the cursor was likewise randomly generated for each new trial. Finally, the extent of the spectrum was jittered one or two stimuli (2.5 or 5 hue steps) from trial to trial, which had the effect of shifting the spectrum slightly in the yellow or the purple direction from trial to trial. This was done to ensure that the blue - green boundary would not fall at a consistent distance from the spectrum endpoints on each trial.
The second two blocks were naming tasks. In each, subjects were shown each of the 27 stimuli of the full range five times in random order, for a total of 135 trials per block. On each trial, subjects were asked to rate how good an example of a given color name each stimulus was. In one block, the color name was green , in the other, the color name was blue ; order of blocks was counterbalanced by subject. To respond, subjects positioned a slider bar with endpoints “Not at all [green/blue]” and “Perfectly [green/blue]” to the desired position matching their judgment of each stimulus, as shown above in Fig 3C . Responses in the naming blocks were self-paced. Naming blocks always followed reconstruction blocks, to ensure that repeated exposure to the color terms green and blue did not bias responses during reconstruction.
The experiment was presented in Matlab version 7.11.0 (R2010b) using Psychtoolbox (version 3) [ 39 – 41 ]. The experiment was conducted in a dark, sound-attenuated booth on an LCD monitor that supported 24-bit color. The monitor had been characterized using a Minolta CS100 colorimeter. A chin rest was used to ensure that each subject viewed the screen from a constant position; when in position, the base of the subject’s chin was situated 30 cm from the screen.
As part of debriefing after testing was complete, each subject was asked to report any strategies they used during the delay block to help them remember the target color. Summaries of each response, as reported by the experimenter, are listed in Table 4 .
When subjects gave specific examples of color terms used as memory aids, they are reported here.
Color spectrum
We wished to consider our stimuli along a 1-dimensional spectrum such that distance between two colors on that spectrum approximates the perceptual difference between those colors. To this end, we first converted our stimuli to CIELAB color space. CIELAB is a 3-dimensional color space designed “in an attempt to provide coordinates for colored stimuli so that the distance between the coordinates of any two stimuli is predictive of the perceived color difference between them” (p. 202 of [ 42 ]). The conversion to CIELAB was done according to the equations on pp. 167-168 of Wyszecki and Stiles (1982) [ 38 ], assuming 2 degree observer and D65 illuminant. For each pair of neighboring colors in the set of 677 colors of our color wheel, we measured the distance (Δ E ) betwen these two colors in CIELAB space. We then arranged all colors along a 1-dimensional spectrum that was scaled to length 1, such that the distance between each pair of neighboring colors along that spectrum was proportional to the CIELAB Δ E distance between them. This CIELAB-based 1-dimensional spectrum was used for our analyses in Study 1, and an analogous spectrum for a different set of colors was used for our analyses in Studies 2 and 3.
Statistical analysis
As a result of the experiment detailed above, we obtained bias data from 20 participants, for each of 19 hues (the medium range), for 5 trials per hue per participant, in each of the simultaneous and delayed conditions. For analysis purposes, we restricted attention to the focused range of stimuli (15 hues), in order to consider a region of the spectrum that is well-centered with respect to green and blue , as we are primarily interested in bias that may be induced by these two categories. We wished to determine whether the magnitude of the bias differed as a function of the simultaneous vs. delayed condition, whether the magnitude of the bias varied as a function of hue, and whether there was an interaction between these two factors. To answer those questions, we conducted a 2 (condition: simultaneous vs. delayed) × 15 (hues) repeated measures analysis of variance (ANOVA), in which the dependent measure was the absolute value of the reproduction bias (reproduced hue minus target hue), averaged across trials for a given participant at a given target hue in a given condition. The ANOVA included an error term to account for across-subject variability. We found a main effect of condition, with greater bias magnitude in the delayed than in the simultaneous condition [ F (1, 19) = 61.61, p < 0.0001], a main effect of hue [ F (14, 266) = 4.565, p < 0.0001], and an interaction of hue and condition [ F (14, 266) = 3.763, p < 0.0001]. All hue calculations were relative to the CIELAB-based spectrum detailed in the preceding section.
We then conducted paired t-tests at each of the target hues, comparing each participant’s bias magnitude for that hue (averaged over trials) in the simultaneous condition vs. the delayed condition. Blue asterisks at the top of Fig 4 mark hues for which the paired t-test returned p < 0.05 when applying Bonferroni corrections for multiple comparisons.
Modeling procedure
We considered four models in accounting for color reconstruction in English speakers: the null model, a 1-category model for which the category was green , a 1-category model for which the category was blue , and a 2-category model based on both green and blue .
We fit these models to the data in two steps. We first fit any category parameters (the means μ c and variances σ c 2 for any categories c ) to the naming data. We then fit the one remaining free parameter ( σ m 2 ), which captures the uncertainty of fine-grained memory, to the bias data, without further adjusting the category parameters. We specify each of these two steps below.
We fit a Gaussian function to the goodness naming data for green , and another Gaussian function to the data for blue , using maximum likelihood estimation. The fitted Gaussian functions can be seen, together with the data to which they were fit, in the top panel of Fig 4 . This process determined values for the category means μ c and category variances σ c 2 for the two categories green and blue .
For each of the four variants of the category adjustment model outlined above (null, 1-category green, 1-category blue, and 2-category), we retained the category parameter settings resulting from the above fit to the naming data. We then obtained a value for the one remaining free parameter σ m 2 , corresponding to the uncertainty of fine-grained memory, by fitting the model to the bias data via maximum likelihood estimation, without further adjusting the category parameters.
Empirical data
The empirical data considered for this study were drawn from two sources: the study of 2AFC color discrimination by speakers of Berinmo and English in Experiment 6a of Roberson et al. (2000) [ 9 ], and the study of 2AFC color discrimination by speakers of Himba and English in Experiment 3b of Roberson et al. (2005) [ 10 ]. In both studies, two sets of color stimuli were considered, all at value (lightness) level 5, and chroma (saturation) level 8. Both sets varied in hue by increments of 2.5 Munsell hue steps. The first set of stimuli was centered at the English green - blue boundary (hue 7.5BG), and contained the following seven hues: 10G, 2.5BG, 5BG, 7.5BG, 10BG, 2.5B, 5B. The second set of stimuli was centered at the Berinmo wor - nol boundary (hue 5GY), and contained the following seven hues: 7.5Y, 10Y, 2.5GY, 5GY, 7.5GY, 10GY, 2.5G. Stimuli in the set that crossed an English category boundary all fell within a single category in Berinmo ( nol ) and in Himba ( burou ), and stimuli in the set that crossed a Berinmo category boundary also crossed a Himba category boundary ( dumbu - burou ) but all fell within a single category in English ( green ), according to naming data in Fig 1 of Roberson et al. (2005) [ 10 ]. Based on specifications in the original empirical studies [ 9 , 10 ], we took the pairs of stimuli probed to be those presented in Table 5 .
Any stimulus pair that includes a boundary color is considered to be a cross-category pair. All hues are at value (lightness) level 5, and chroma (saturation) level 8. 1s denotes a 1-step pair; 2s denotes a 2-step pair.
Based on naming data in Fig 1 of Roberson et al. 2005 [ 10 ], we took the prototypes of the relevant color terms to be:
English green prototype = 10GY
English blue prototype = 10B
Berinmo wor prototype = 5Y
Berinmo nol prototype = 5G
Himba dumbu prototype = 5Y
Himba burou prototype = 10G
Fig 6 above shows a spectrum of hues ranging from the Berinmo wor prototype (5Y) to the English blue prototype (10B) in increments of 2.5 Munsell hue steps, categorized according to each of the three languages we consider here. These Munsell hues were converted to xyY and then to CIELAB as above, and the positions of the hues on the spectrum were adjusted so that the distance between each two neighboring hues in the spectrum is proportional to the CIELAB Δ E distance between them. We use this CIELAB-based spectrum for our analyses below. The two shaded regions on each spectrum in Fig 6 denote the two target sets of stimuli identified above.
The discrimination data we modeled were drawn from Table 11 of Roberson et al. (2000:392) [ 9 ] and Table 6 of Roberson et al. (2005:400) [ 10 ].
We considered three variants of the 2-category model: an English blue - green model, a Berinmo wor - nol model, and a Himba dumbu - burou model. As in Study 1, we fit each model to the data in two steps. For each language’s model, we first fit the category component of that model to naming data from that language. Because color naming differs across these languages, this resulted in three models with different category components. For each model, we then retained and fixed the resulting category parameter settings, and fit the single remaining parameter, corresponding to memory uncertainty, to discrimination data. We detail these two steps below.
For the naming data, we modeled the probability of applying category name c to stimulus i as:
where p ( c ) is assumed to be uniform, and f ( i | c ) is a non-normalized Gaussian function corresponding to category c , with mean μ c and variance σ c 2 . There were two categories c for each model, e.g. wor and nol in the case of the Berinmo model. Category means μ c were set to the corresponding category prototypes shown above (e.g. μ c for Berinmo nol corresponded to 5G), and category variances σ c 2 were left as free parameters. We then adjusted these free category variances to reproduce the empirical boundary between the two categories c 1 and c 2 for that language, as follows. Sweeping from left ( c 1 ) to right ( c 2 ), we took the model’s boundary between c 1 and c 2 to be the first position i on the spectrum for which p ( c 1 | i ) ≤ p ( c 2 | i ); we refer to this as the model crossover point . We measured the distance in the CIELAB-based spectrum between the model crossover point and the empirical category boundary, and adjusted the category variances σ c 1 2 and σ c 2 2 so as to minimize that distance. This was done separately for each language’s model. Fig 6 shows the resulting fits of category components to naming data for each of the three languages.
We then simulated performance in the 2AFC discrimination task for each stimulus pair in Table 5 , by each model, as follows. Given a pair of stimuli, one stimulus was taken to be the target t and therefore held in memory, and the other taken to be the distractor d . We took the reconstruction r for the target stimulus t to be the expected value of the posterior for the 2-category model:
We then measured, along the hue spectrum in question, the distance dist ( r , t ) between the reconstruction r and the target t , and the distance dist ( r , d ) between the reconstruction r and the distractor d . We converted each of these two distances to a similarity score:
and modeled the proportion correct choice as:
These equations are based on Luce’s [ 43 ] (pp. 113-114) model of choice behavior. For each pair of stimuli, each stimulus was once taken to be the target, and once taken to be the distractor, and the results averaged to yield a mean discrimination score for that pair. Scores were then averaged across all pairs listed as within-category pairs, and separately for all pairs listed as cross-category pairs. These scores were range-matched to the empirical data, in an attempt to correct for other factors that could affect performance, such as familiarity with such tasks, etc.; such external factors could in principle differ substantially across participant pools for the three languages modeled. We measured MSE between the model output and the data so treated, and adjusted the remaining parameter σ m 2 , corresponding to memory uncertainty, so as to minimize this MSE. This entire process was conducted two times. The first time, each language’s model was fit to that same language’s discrimination data. Then, to test whether native-language categories allow a better fit than the categories of another language, we fit the Berinmo model to the English discrimination data (and vice versa), and the Himba model to the English discrimination data (and vice versa).
The empirical data considered for this study are those of Figs 2 (English green/blue , 10 second delay), 3 (Berinmo wor/nol ), and 4 (Himba dumbu/borou ) of Hanley and Roberson (2011) [ 36 ]. These data were originally published by Roberson and Davidoff (2000) [ 8 ], Roberson et al. (2000) [ 9 ], and Roberson et al. (2005) [ 10 ], respectively. The Berinmo and Himba stimuli and data were the same as in our Study 2, but the English stimuli and data reanalyzed by Hanley and Roberson (2011) [ 36 ] Fig 2 were instead drawn from Table 1 of Roberson and Davidoff (2000) [ 8 ], reproduced here in Table 6 , and used for the English condition of this study. These stimuli for English were at lightness (value) level 4, rather than 5 as for the other two languages. We chose to ignore this difference for modeling purposes.
Any stimulus pair that includes a boundary color is considered to be a cross-category pair. All hues are at value (lightness) level 4, and chroma (saturation) level 8. 1s denotes a 1-step pair; 2s denotes a 2-step pair.
All modeling procedures were identical to those of Study 2, with the exception that GE (target = good exemplar) and PE (target = poor exemplar) cases were disaggregated, and analyzed separately.
Acknowledgments
We thank Roland Baddeley, Paul Kay, Charles Kemp, Steven Piantadosi, and an anonymous reviewer for their comments.
Funding Statement
This research was supported by the National Science Foundation ( www.nsf.gov ) under grants DGE-1106400 (EC) and SBE-1041707 (YX, TR). Publication was made possible in part by support from the Berkeley Research Impact Initiative (BRII) sponsored by the UC Berkeley Library. The funders had no role in study design, data collection and analysis, decision to publish, or preparation of the manuscript.
Data Availability
- Bipolar Disorder
- Therapy Center
- When To See a Therapist
- Types of Therapy
- Best Online Therapy
- Best Couples Therapy
- Best Family Therapy
- Managing Stress
- Sleep and Dreaming
- Understanding Emotions
- Self-Improvement
- Healthy Relationships
- Student Resources
- Personality Types
- Guided Meditations
- Verywell Mind Insights
- 2023 Verywell Mind 25
- Mental Health in the Classroom
- Editorial Process
- Meet Our Review Board
- Crisis Support
The Sapir-Whorf Hypothesis: How Language Influences How We Express Ourselves
Rachael is a New York-based writer and freelance writer for Verywell Mind, where she leverages her decades of personal experience with and research on mental illness—particularly ADHD and depression—to help readers better understand how their mind works and how to manage their mental health.
:max_bytes(150000):strip_icc():format(webp)/RachaelGreenProfilePicture1-a3b8368ef3bb47ccbac92c5cc088e24d.jpg)
Thomas Barwick / Getty Images
What to Know About the Sapir-Whorf Hypothesis
Real-world examples of linguistic relativity, linguistic relativity in psychology.
The Sapir-Whorf Hypothesis, also known as linguistic relativity, refers to the idea that the language a person speaks can influence their worldview, thought, and even how they experience and understand the world.
While more extreme versions of the hypothesis have largely been discredited, a growing body of research has demonstrated that language can meaningfully shape how we understand the world around us and even ourselves.
Keep reading to learn more about linguistic relativity, including some real-world examples of how it shapes thoughts, emotions, and behavior.
The hypothesis is named after anthropologist and linguist Edward Sapir and his student, Benjamin Lee Whorf. While the hypothesis is named after them both, the two never actually formally co-authored a coherent hypothesis together.
This Hypothesis Aims to Figure Out How Language and Culture Are Connected
Sapir was interested in charting the difference in language and cultural worldviews, including how language and culture influence each other. Whorf took this work on how language and culture shape each other a step further to explore how different languages might shape thought and behavior.
Since then, the concept has evolved into multiple variations, some more credible than others.
Linguistic Determinism Is an Extreme Version of the Hypothesis
Linguistic determinism, for example, is a more extreme version suggesting that a person’s perception and thought are limited to the language they speak. An early example of linguistic determinism comes from Whorf himself who argued that the Hopi people in Arizona don’t conjugate verbs into past, present, and future tenses as English speakers do and that their words for units of time (like “day” or “hour”) were verbs rather than nouns.
From this, he concluded that the Hopi don’t view time as a physical object that can be counted out in minutes and hours the way English speakers do. Instead, Whorf argued, the Hopi view time as a formless process.
This was then taken by others to mean that the Hopi don’t have any concept of time—an extreme view that has since been repeatedly disproven.
There is some evidence for a more nuanced version of linguistic relativity, which suggests that the structure and vocabulary of the language you speak can influence how you understand the world around you. To understand this better, it helps to look at real-world examples of the effects language can have on thought and behavior.
Different Languages Express Colors Differently
Color is one of the most common examples of linguistic relativity. Most known languages have somewhere between two and twelve color terms, and the way colors are categorized varies widely. In English, for example, there are distinct categories for blue and green .
Blue and Green
But in Korean, there is one word that encompasses both. This doesn’t mean Korean speakers can’t see blue, it just means blue is understood as a variant of green rather than a distinct color category all its own.
In Russian, meanwhile, the colors that English speakers would lump under the umbrella term of “blue” are further subdivided into two distinct color categories, “siniy” and “goluboy.” They roughly correspond to light blue and dark blue in English. But to Russian speakers, they are as distinct as orange and brown .
In one study comparing English and Russian speakers, participants were shown a color square and then asked to choose which of the two color squares below it was the closest in shade to the first square.
The test specifically focused on varying shades of blue ranging from “siniy” to “goluboy.” Russian speakers were not only faster at selecting the matching color square but were more accurate in their selections.
The Way Location Is Expressed Varies Across Languages
This same variation occurs in other areas of language. For example, in Guugu Ymithirr, a language spoken by Aboriginal Australians, spatial orientation is always described in absolute terms of cardinal directions. While an English speaker would say the laptop is “in front of” you, a Guugu Ymithirr speaker would say it was north, south, west, or east of you.
As a result, Aboriginal Australians have to be constantly attuned to cardinal directions because their language requires it (just as Russian speakers develop a more instinctive ability to discern between shades of what English speakers call blue because their language requires it).
So when you ask a Guugu Ymithirr speaker to tell you which way south is, they can point in the right direction without a moment’s hesitation. Meanwhile, most English speakers would struggle to accurately identify South without the help of a compass or taking a moment to recall grade school lessons about how to find it.
The concept of these cardinal directions exists in English, but English speakers aren’t required to think about or use them on a daily basis so it’s not as intuitive or ingrained in how they orient themselves in space.
Just as with other aspects of thought and perception, the vocabulary and grammatical structure we have for thinking about or talking about what we feel doesn’t create our feelings, but it does shape how we understand them and, to an extent, how we experience them.
Words Help Us Put a Name to Our Emotions
For example, the ability to detect displeasure from a person’s face is universal. But in a language that has the words “angry” and “sad,” you can further distinguish what kind of displeasure you observe in their facial expression. This doesn’t mean humans never experienced anger or sadness before words for them emerged. But they may have struggled to understand or explain the subtle differences between different dimensions of displeasure.
In one study of English speakers, toddlers were shown a picture of a person with an angry facial expression. Then, they were given a set of pictures of people displaying different expressions including happy, sad, surprised, scared, disgusted, or angry. Researchers asked them to put all the pictures that matched the first angry face picture into a box.
The two-year-olds in the experiment tended to place all faces except happy faces into the box. But four-year-olds were more selective, often leaving out sad or fearful faces as well as happy faces. This suggests that as our vocabulary for talking about emotions expands, so does our ability to understand and distinguish those emotions.
But some research suggests the influence is not limited to just developing a wider vocabulary for categorizing emotions. Language may “also help constitute emotion by cohering sensations into specific perceptions of ‘anger,’ ‘disgust,’ ‘fear,’ etc.,” said Dr. Harold Hong, a board-certified psychiatrist at New Waters Recovery in North Carolina.
As our vocabulary for talking about emotions expands, so does our ability to understand and distinguish those emotions.
Words for emotions, like words for colors, are an attempt to categorize a spectrum of sensations into a handful of distinct categories. And, like color, there’s no objective or hard rule on where the boundaries between emotions should be which can lead to variation across languages in how emotions are categorized.
Emotions Are Categorized Differently in Different Languages
Just as different languages categorize color a little differently, researchers have also found differences in how emotions are categorized. In German, for example, there’s an emotion called “gemütlichkeit.”
While it’s usually translated as “cozy” or “ friendly ” in English, there really isn’t a direct translation. It refers to a particular kind of peace and sense of belonging that a person feels when surrounded by the people they love or feel connected to in a place they feel comfortable and free to be who they are.
Harold Hong, MD, Psychiatrist
The lack of a word for an emotion in a language does not mean that its speakers don't experience that emotion.
You may have felt gemütlichkeit when staying up with your friends to joke and play games at a sleepover. You may feel it when you visit home for the holidays and spend your time eating, laughing, and reminiscing with your family in the house you grew up in.
In Japanese, the word “amae” is just as difficult to translate into English. Usually, it’s translated as "spoiled child" or "presumed indulgence," as in making a request and assuming it will be indulged. But both of those have strong negative connotations in English and amae is a positive emotion .
Instead of being spoiled or coddled, it’s referring to that particular kind of trust and assurance that comes with being nurtured by someone and knowing that you can ask for what you want without worrying whether the other person might feel resentful or burdened by your request.
You might have felt amae when your car broke down and you immediately called your mom to pick you up, without having to worry for even a second whether or not she would drop everything to help you.
Regardless of which languages you speak, though, you’re capable of feeling both of these emotions. “The lack of a word for an emotion in a language does not mean that its speakers don't experience that emotion,” Dr. Hong explained.
What This Means For You
“While having the words to describe emotions can help us better understand and regulate them, it is possible to experience and express those emotions without specific labels for them.” Without the words for these feelings, you can still feel them but you just might not be able to identify them as readily or clearly as someone who does have those words.
Rhee S. Lexicalization patterns in color naming in Korean . In: Raffaelli I, Katunar D, Kerovec B, eds. Studies in Functional and Structural Linguistics. Vol 78. John Benjamins Publishing Company; 2019:109-128. Doi:10.1075/sfsl.78.06rhe
Winawer J, Witthoft N, Frank MC, Wu L, Wade AR, Boroditsky L. Russian blues reveal effects of language on color discrimination . Proc Natl Acad Sci USA. 2007;104(19):7780-7785. 10.1073/pnas.0701644104
Lindquist KA, MacCormack JK, Shablack H. The role of language in emotion: predictions from psychological constructionism . Front Psychol. 2015;6. Doi:10.3389/fpsyg.2015.00444
By Rachael Green Rachael is a New York-based writer and freelance writer for Verywell Mind, where she leverages her decades of personal experience with and research on mental illness—particularly ADHD and depression—to help readers better understand how their mind works and how to manage their mental health.
Click through the PLOS taxonomy to find articles in your field.
For more information about PLOS Subject Areas, click here .
Loading metrics
Open Access
Peer-reviewed
Research Article
The Sapir-Whorf Hypothesis and Probabilistic Inference: Evidence from the Domain of Color
Contributed equally to this work with: Emily Cibelli, Yang Xu
Affiliation Department of Linguistics, Northwestern University, Evanston, IL 60208, United States of America
Affiliations Department of Linguistics, University of California, Berkeley, CA 94720, United States of America, Cognitive Science Program, University of California, Berkeley, CA 94720, United States of America
Affiliation Department of Psychology, University of Wisconsin, Madison, WI 53706, United States of America
Affiliations Cognitive Science Program, University of California, Berkeley, CA 94720, United States of America, Department of Psychology, University of California, Berkeley, CA 94720, United States of America
* E-mail: [email protected]
- Emily Cibelli,
- Yang Xu,
- Joseph L. Austerweil,
- Thomas L. Griffiths,
- Terry Regier
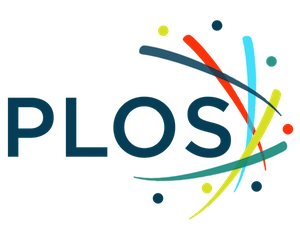
- Published: July 19, 2016
- https://doi.org/10.1371/journal.pone.0158725
- Reader Comments
16 Aug 2016: The PLOS ONE Staff (2016) Correction: The Sapir-Whorf Hypothesis and Probabilistic Inference: Evidence from the Domain of Color. PLOS ONE 11(8): e0161521. https://doi.org/10.1371/journal.pone.0161521 View correction
The Sapir-Whorf hypothesis holds that our thoughts are shaped by our native language, and that speakers of different languages therefore think differently. This hypothesis is controversial in part because it appears to deny the possibility of a universal groundwork for human cognition, and in part because some findings taken to support it have not reliably replicated. We argue that considering this hypothesis through the lens of probabilistic inference has the potential to resolve both issues, at least with respect to certain prominent findings in the domain of color cognition. We explore a probabilistic model that is grounded in a presumed universal perceptual color space and in language-specific categories over that space. The model predicts that categories will most clearly affect color memory when perceptual information is uncertain. In line with earlier studies, we show that this model accounts for language-consistent biases in color reconstruction from memory in English speakers, modulated by uncertainty. We also show, to our knowledge for the first time, that such a model accounts for influential existing data on cross-language differences in color discrimination from memory, both within and across categories. We suggest that these ideas may help to clarify the debate over the Sapir-Whorf hypothesis.
Citation: Cibelli E, Xu Y, Austerweil JL, Griffiths TL, Regier T (2016) The Sapir-Whorf Hypothesis and Probabilistic Inference: Evidence from the Domain of Color. PLoS ONE 11(7): e0158725. https://doi.org/10.1371/journal.pone.0158725
Editor: Daniel Osorio, University of Sussex, UNITED KINGDOM
Received: October 26, 2015; Accepted: June 21, 2016; Published: July 19, 2016
Copyright: © 2016 Cibelli et al. This is an open access article distributed under the terms of the Creative Commons Attribution License , which permits unrestricted use, distribution, and reproduction in any medium, provided the original author and source are credited.
Data Availability: All relevant data are available within the paper and/or at: https://github.com/yangxuch/probwhorfcolor This GitHub repository is mentioned in the paper.
Funding: This research was supported by the National Science Foundation ( www.nsf.gov ) under grants DGE-1106400 (EC) and SBE-1041707 (YX, TR). Publication was made possible in part by support from the Berkeley Research Impact Initiative (BRII) sponsored by the UC Berkeley Library. The funders had no role in study design, data collection and analysis, decision to publish, or preparation of the manuscript.
Competing interests: The authors have declared that no competing interests exist.
Introduction
The Sapir-Whorf hypothesis [ 1 , 2 ] holds that our thoughts are shaped by our native language, and that speakers of different languages therefore think about the world in different ways. This proposal has been controversial for at least two reasons, both of which are well-exemplified in the semantic domain of color. The first source of controversy is that the hypothesis appears to undercut any possibility of a universal foundation for human cognition. This idea sits uneasily with the finding that variation in color naming across languages is constrained, such that certain patterns of color naming recur frequently across languages [ 3 – 5 ], suggesting some sort of underlying universal basis. The second source of controversy is that while some findings support the hypothesis, they do not always replicate reliably. Many studies have found that speakers of a given language remember and process color in a manner that reflects the color categories of their language [ 6 – 13 ]. Reinforcing the idea that language is implicated in these findings, it has been shown that the apparent effect of language on color cognition disappears when participants are given a verbal [ 7 ] (but not a visual) interference task [ 8 , 11 , 12 ]; this suggests that language may operate through on-line use of verbal representations that can be temporarily disabled. However, some of these findings have a mixed record of replication [ 14 – 17 ]. Thus, despite the substantial empirical evidence already available, the role of language in color cognition remains disputed.
An existing theoretical stance holds the potential to resolve both sources of controversy. On the one hand, it explains effects of language on cognition in a framework that retains a universal component, building on a proposal by Kay and Kempton [ 7 ]. On the other hand, it has the potential to explain when effects of language on color cognition will appear, and when they will not—and why. This existing stance is that of the “category adjustment” model of Huttenlocher and colleagues [ 18 , 19 ]. We adopt this stance, and cast color memory as inference under uncertainty, instantiated in a category adjustment model, following Bae et al. [ 20 ] and Persaud and Hemmer [ 21 ]. The model holds that color memory involves the probabilistic combination of evidence from two sources: a fine-grained representation of the particular color seen, and the language-specific category in which it fell (e.g. English green ). Both sources of evidence are represented in a universal perceptual color space, yet their combination yields language-specific bias patterns in memory, as illustrated in Fig 1 . The model predicts that such category effects will be strongest when fine-grained perceptual information is uncertain. It thus has the potential to explain the mixed pattern of replications of Whorfian effects in the literature: non-replications could be the result of high perceptual certainty.
- PPT PowerPoint slide
- PNG larger image
- TIFF original image
A stimulus is encoded in two ways: (1) a fine-grained representation of the stimulus itself, shown as a (gray) distribution over stimulus space centered at the stimulus’ location in that space, and (2) the language-specific category (e.g. English “green”) in which the stimulus falls, shown as a separate (green) distribution over the same space, centered at the category prototype. The stimulus is reconstructed by combining these two sources of information through probabilistic inference, resulting in a reconstruction of the stimulus (black distribution) that is biased toward the category prototype. Adapted from Fig 11 of Bae et al. (2015) [ 20 ].
https://doi.org/10.1371/journal.pone.0158725.g001
In the category adjustment model, both the fine-grained representation of the stimulus and the category in which it falls are modeled as probability distributions over a universal perceptual color space. The fine-grained representation is veridical (unbiased) but inexact: its distribution is centered at the location in color space where the stimulus itself fell, and the variance of that distribution captures the observer’s uncertainty about the precise location of the stimulus in color space, with greater variance corresponding to greater uncertainty. Psychologically, such uncertainty might be caused by noise in perception itself, by memory decay over time, or by some other cause—and any increase in such uncertainty is modeled by a wider, flatter distribution for the fine-grained representation. The category distribution, in contrast, captures the information about stimulus location that is given by the named category in which the stimulus fell (e.g. green for an English-speaking observer). Because named color categories vary across languages, this category distribution is assumed to be language-specific—although the space over which it exists is universal. The model infers the original stimulus location by combining evidence from both of these distributions. As a result, the model tends to produce reconstructions of the stimulus that are biased away from the actual location of the stimulus and toward the prototype of the category in which it falls.
As illustrated in Fig 2 , this pattern of bias pulls stimuli on opposite sides of a category boundary in opposite directions, producing enhanced distinctiveness for such stimuli. Such enhanced distinctiveness across a category boundary is the signature of categorical perception, or analogous category effects in memory. On this view, language-specific effects on memory can emerge from a largely universal substrate when one critical component of that substrate is language-specific: the category distribution.
Model reconstructions tend to be biased toward category prototypes, yielding enhanced distinctiveness for two stimuli that fall on different sides of a category boundary. Categories are shown as distributions in green and blue; stimuli are shown as vertical black lines; reconstruction bias patterns are shown as arrows.
https://doi.org/10.1371/journal.pone.0158725.g002
If supported, the category adjustment model holds the potential to clarify the debate over the Sapir-Whorf hypothesis in three ways. First, it would link that debate to independent principles of probabilistic inference. In so doing, it would underscore the potentially important role of uncertainty , whether originating in memory or perception, in framing the debate theoretically. Second, and relatedly, it would suggest a possible reason why effects of language on color memory and perception are sometimes found, and sometimes not [ 17 ]. Concretely, the model predicts that greater uncertainty in the fine-grained representation—induced for example through a memory delay, or noise in perception—will lead to greater influence of the category, and thus a stronger bias in reproduction. The mirror-image of this prediction is that in situations of relatively high certainty in memory or perception, there will be little influence of the category, to the point that such an influence may not be empirically detectable. Third, the model suggests a way to think about the Sapir-Whorf hypothesis without jettisoning the important idea of a universal foundation for cognition.
Closely related ideas appear in the literature on probabilistic cue integration [ 22 – 25 ]. For example, Ernst and Banks [ 24 ] investigated perceptual integration of cues from vision and touch in judging the height of an object. They found that humans integrate visual and haptic cues in a statistically optimal fashion, modulated by cue certainty. The category adjustment model we explore here can be seen as a form of probabilistic cue integration in which one of the cues is a language-specific category.
The category adjustment model has been used to account for category effects in various domains, including spatial location [ 18 , 26 ], object size [ 19 , 27 ], and vowel perception [ 28 ]. The category adjustment model also bears similarities to other theoretical accounts of the Sapir-Whorf hypothesis that emphasize the importance of verbal codes [ 7 , 8 ], and the interplay of such codes with perceptual representations [ 29 – 31 ]. Prior research has linked such category effects to probabilistic inference, following the work of Huttenlocher and colleagues [ 18 , 19 ]. Roberson and colleagues [ 32 ] invoked the category adjustment model as a possible explanation for categorical perception of facial expressions, but did not explore a formal computational model; Goldstone [ 33 ] similarly referenced the category adjustment model with respect to category effects in the color domain. Persaud and Hemmer [ 21 , 34 ] explored bias in memory for color, and compared empirically obtained memory bias patterns from English speakers with results predicted by a formally specified category adjustment model, but did not link those results to the debate over the Sapir-Whorf hypothesis, and did not manipulate uncertainty. More recently, a subsequent paper by the same authors and colleagues [ 35 ] explored category-induced bias in speakers of another language, Tsimané, and did situate those results with respect to the Sapir-Whorf hypothesis, but again did not manipulate uncertainty. Most recently, Bae et al. [ 20 ] extensively documented bias in color memory in English speakers, modeled those results with a category-adjustment computational model, and did manipulate uncertainty—but did not explore these ideas relative to the Sapir-Whorf hypothesis, or to data from different languages.
In what follows, we first present data and computational simulations that support the recent finding that color memory in English speakers is well-predicted by a category adjustment model, with the strength of category effects modulated by uncertainty. We then show, to our knowledge for the first time, that a category adjustment model accounts for influential existing cross-language data on color that support the Sapir-Whorf hypothesis.
In this section we provide general descriptions of our analyses and results. Full details are supplied in the section on Materials and Methods.
Study 1: Color reconstruction in English speakers
Our first study tests the core assumptions of the category adjustment model in English speakers. In doing so, it probes questions that were pursued by two studies that appeared recently, after this work had begun. Persaud and Hemmer [ 21 ] and Bae et al. [ 20 ] both showed that English speakers’ memory for a color tends to be biased toward the category prototype of the corresponding English color term, in line with a category adjustment model. Bae et al. [ 20 ] also showed that the amount of such bias increases when subjects must retain the stimulus in memory during a delay period, compared to when there is no such delay, as predicted by the principles of the category adjustment model. In our first study, we consider new evidence from English speakers that tests these questions, prior to considering speakers of different languages in our following studies.
English-speaking participants viewed a set of hues that varied in small steps from dark yellow to purple, with most hues corresponding to some variety of either green or blue. We collected two kinds of data from these participants: bias data and naming data. Bias data were based on participants’ non-linguistic reconstruction of particular colors seen. Specifically, for each hue seen, participants recreated that hue by selecting a color from a color wheel, either while the target was still visible ( Fig 3A : simultaneous condition), or from memory after a short delay ( Fig 3B : delayed condition). We refer to the resulting data as bias data, because we are interested in the extent to which participants’ reconstructions of the stimulus color are biased away from the original target stimulus. Afterwards, the same participants indicated how good an example of English green (as in Fig 3C ) and how good an example of English blue each hue was. We refer to these linguistic data as naming data.
Screenshots of example trials illustrating (A) simultaneous reconstruction, (B) delayed reconstruction, and (C) green goodness rating.
https://doi.org/10.1371/journal.pone.0158725.g003
Fig 4 shows both naming and bias data as a function of target hue. The top panel of the figure shows the naming data and also shows Gaussian functions corresponding to the English color terms green and blue that we fitted to the naming data. Bias data were collected for only a subset of the hues for which naming data were collected, and the shaded region in the top panel of Fig 4 shows that subset, relative to the full range of hues for naming data. We collected bias data only in this smaller range because we were interested specifically in bias induced by the two color terms blue and green , and colors outside the shaded region seemed to us to clearly show some influence of neighboring categories such as yellow and purple . The bottom panel of the figure shows the bias data, plotted relative to the prototypes (means) of the fitted Gaussian functions for green and blue . It can be seen that reconstruction bias appears to be stronger in the delayed than in the simultaneous condition, as predicted, and that—especially in the delayed condition—there is an inflection in the bias pattern between the two category prototypes, suggesting that bias may reflect the influence of each of the two categories. The smaller shaded region in this bottom panel denotes the subset of these hues that we subsequently analyzed statistically, and to which we fit models. We reduced the range of considered hues slightly further at this stage, to ensure that the range was well-centered with respect to the two relevant category prototypes, for green and blue , as determined by the naming data.
In both top and bottom panels, the horizontal axis denotes target hue, ranging from yellow on the left to purple on the right. Top panel (naming data): The solid green and blue curves show, for each target hue, the average goodness rating for English green and blue respectively, as a proportion of the maximum rating possible. The dashed green and blue curves show Gaussian functions fitted to the naming goodness data. The dotted vertical lines marked at the bottom with green and blue squares denote the prototypes for green and blue , determined as the means of the green and blue fitted Gaussian functions, respectively. The shaded region in the top panel shows the portion of the spectrum for which bias data were collected. Bottom panel (bias data): Solid curves denote, for each target hue, the average reconstruction bias for that hue, such that positive values denote reconstruction bias toward the purple (here, right) end of the spectrum, and negative values denote reconstruction bias toward the yellow (here, left) end of the spectrum. Units for the vertical axis are the same as for the horizontal axis, which is normalized to length 1.0. The black and red curves show bias under simultaneous and delayed response, respectively. Blue stars at the top of the bottom panel mark hues for which there was a significant difference in the magnitude of bias between simultaneous and delayed conditions. The shaded region in the bottom panel shows the portion of the data that was analyzed statistically, and to which models were fit. In both panels, error bars represent standard error of the mean.
https://doi.org/10.1371/journal.pone.0158725.g004
The absolute values (magnitudes) of the bias were analyzed using a 2 (condition: simultaneous vs. delayed) × 15 (hues) repeated measures analysis of variance. This analysis revealed significantly greater bias magnitude in the delayed than in the simultaneous condition. It also revealed that bias magnitude differed significantly as a function of hue, as well as a significant interaction between the factors of hue and condition. The blue stars in Fig 4 denote hues for which the difference in bias magnitude between the simultaneous and delayed conditions reached significance. The finding of greater bias magnitude in the delayed than in the simultaneous condition is consistent with the proposal that uncertainty is an important mediating factor in such category effects, as argued by Bae et al. [ 20 ]. It also suggests that some documented failures to find such category effects could in principle be attributable to high certainty, a possibility that can be explored by manipulating uncertainty.
We wished to test in a more targeted fashion to what extent these data are consistent with a category adjustment model in which a color is reconstructed based in part on English named color categories. To that end, we compared the performance of four models against these data; only one of these models considered both of the relevant English color categories, green and blue . As in Fig 1 , each model contains a fine-grained but inexact representation of the perceived stimulus, and (for most models) a representation of one or more English color categories. Each model predicts the reconstruction of the target stimulus from its fine-grained representation of the target together with any category information. Category information in the model is specified by the naming data. Each model has a single free parameter, corresponding to the uncertainty of the fine-grained representation; this parameter is fit to bias data.
- The null model is a baseline model that predicts hue reconstruction based only on the fine-grained representation of the stimulus, with no category component.
- The 1-category (green) model predicts hue reconstruction based on the fine-grained representation of the stimulus, combined with a representation of only the green category, derived from the green naming data.
- The 1-category (blue) model predicts hue reconstruction based on the fine-grained representation of the stimulus, combined with a representation of only the blue category, derived from the blue naming data.
- The 2-category model predicts hue reconstruction based on the fine-grained representation of the stimulus, combined with representations of both the green and blue categories.
If reproduction bias reflects probabilistic inference from a fine-grained representation of the stimulus itself, together with any relevant category, we would expect the 2-category model to outperform the others. The other models have access either to no category information at all (null model), or to category information for only one of the two relevant color categories (only one of green and blue ). The 2-category model in contrast combines fine-grained stimulus information with both of the relevant categories ( green and blue ); this model thus corresponds most closely to a full category adjustment model.
Fig 5 redisplays the data from simultaneous and delayed reconstruction, this time with model fits overlaid. The panels in the left column show data from simultaneous reconstruction, fit by each of the four models, and the panels in the right column analogously show data and model fits from delayed reconstruction. Visually, it appears that in the case of delayed reconstruction, the 2-category model fits the data at least qualitatively better than competing models: it shows an inflection in bias as the empirical data do, although not as strongly. For simultaneous reconstruction, the 2-category model fit is also reasonable but visually not as clearly superior to the others (especially the null model) as in the delayed condition.
Left column: Bias from simultaneous reconstruction, fit by each of the four models. The empirical data (black lines with error bars) in these four panels are the same, and only the model fits (red lines) differ. Within each panel, the horizontal axis denotes target hue, and the vertical axis denotes reconstruction bias. The green and blue prototypes are indicated as vertical lines with green and blue squares at the bottom. Right column: delayed reconstruction, displayed analogously.
https://doi.org/10.1371/journal.pone.0158725.g005
Table 1 reports quantitative results of these model fits. The best fit is provided by the 2-category model, in both the simultaneous and delayed conditions, whether assessed by log likelihood (LL) or by mean squared errror (MSE). In line with earlier studies [ 20 , 21 ], these findings demonstrate that a category adjustment model that assumes stimulus reconstruction is governed by relevant English color terms provides a reasonable fit to data on color reconstruction by English speakers. The category adjustment model fits well both when the category bias is relatively slight (simultaneous condition), and when the bias is stronger (delayed condition).
LL = log likelihood (higher is better). MSE = mean squared error (lower is better). The best value in each row is shown in bold .
https://doi.org/10.1371/journal.pone.0158725.t001
Study 2: Color discrimination across languages
The study above examined the categories of just one language, English, whereas the Sapir-Whorf hypothesis concerns cross-language differences in categorization, and their effect on cognition and perception. Empirical work concerning this hypothesis has not specifically emphasized bias in reconstruction, but there is a substantial amount of cross-language data of other sorts against which the category adjustment model can be assessed. One method that has been extensively used to explore the Sapir-Whorf hypothesis in the domain of color is a two-alternative forced choice (2AFC) task. In such a task, participants first are briefly shown a target color, and then shortly afterward are shown that same target color together with a different distractor color, and are asked to indicate which was the color originally seen. A general finding from such studies [ 8 – 10 ] is that participants exhibit enhanced discrimination for pairs of colors that would be named differently in their native language. For example, in such a 2AFC task, speakers of English show enhanced discrimination for colors from the different English categories green and blue , compared with colors from the same category (either both green or both blue ) [ 8 ]. In contrast, speakers of the Berinmo language, which has named color categories that differ from those of English, show enhanced discrimination across Berinmo category boundaries, and not across those of English [ 9 ]. Thus color discrimination in this task is enhanced at the boundaries of native language categories, suggesting an effect of those native language categories on the ability to discriminate colors from memory.
Considered informally, this qualitative pattern of results appears to be consistent with the category adjustment model, as suggested above in Fig 2 . We wished to determine whether such a model would also provide a good quantitative account of such results, when assessed using the specific color stimuli and native-language naming patterns considered in the empirical studies just referenced.
We considered cross-language results from two previous studies by Debi Roberson and colleagues, one that compared color memory in speakers of English and Berinmo, a language of Papua New Guinea [ 9 ], and another that explored color memory in speakers of Himba, a language of Namibia [ 10 ]. Berinmo and Himba each have five basic color terms, in contrast with eleven in English. The Berinmo and Himba color category systems are similar to each other in broad outline, but nonetheless differ noticeably. Following these two previous studies, we considered the following pairs of categories in these three languages:
- the English categories green and blue ,
- the Berinmo categories wor (covering roughly yellow, orange, and brown), and nol (covering roughly green, blue, and purple), and
- the Himba categories dumbu (covering roughly yellow and beige) and burou (covering roughly green, blue, and purple).
These three pairs of categories are illustrated in Fig 6 , using naming data from Roberson et al. (2000) [ 9 ] and Roberson et al. (2005) [ 10 ]. It can be seen that the English green - blue distinction is quite different from the Berinmo wor - nol and the Himba dumbu - burou distinctions, which are similar but not identical to each other. The shaded regions in this figure indicate specific colors that were probed in discrimination tasks. The shaded (probed) region that straddles a category boundary in Berinmo and Himba falls entirely within the English category green , and the shaded (probed) region that straddles a category boundary in English falls entirely within the Berinmo category nol and the Himba category burou , according to naming data in Fig 1 of Roberson et al. (2005) [ 10 ]. The empirical discrimination data in Fig 7 are based on those probed colors [ 9 , 10 ], and show that in general, speakers of a language tend to exhibit greater discrimination for pairs of colors that cross a category boundary in their native language, consistent with the Sapir-Whorf hypothesis.
The English categories green and blue (top panel), the Berinmo categories wor and nol (middle panel), and the Himba categories dumbu and burou (bottom panel), plotted against a spectrum of hues that ranges from dark yellow at the left, through green, to blue at the right. Colored squares mark prototypes: the shared prototype for Berinmo wor and Himba dumbu , and the prototypes for English green and blue ; the color of each square approximates the color of the corresponding prototype. For each language, the dotted-and-dashed vertical lines denote the prototypes for the two categories from that language, and the dashed vertical line denotes the empirical boundary between these two categories. Black curves show the probability of assigning a given hue to each of the two native-language categories, according to the category component of a 2-category model fit to each language’s naming data. The shaded regions mark the ranges of colors probed in discrimination tasks; these two regions are centered at the English green - blue boundary and the Berinmo wor - nol boundary. Data are from Roberson et al. (2000) [ 9 ] and Roberson et al. (2005) [ 10 ].
https://doi.org/10.1371/journal.pone.0158725.g006
Top panels: Discrimination from memory by Berinmo and English speakers for pairs of colors across and within English and Berinmo color category boundaries. Empirical data are from Table 11 of Roberson et al. (2000:392). Empirical values show mean proportion correct 2AFC memory judgments, and error bars show standard error. Model values show mean model proportion correct 2AFC memory judgments after simulated reconstruction with native-language categories. Model results are range-matched to the corresponding empirical values, such that the minimum and maximum model values match the minimum and maximum mean values in the corresponding empirical dataset, and other model values are linearly interpolated. Bottom panels: Discrimination from memory by Himba and English speakers for pairs of colors across and within English and Himba color category boundaries, compared with model results based on native-language categories. Empirical data are from Table 6 of Roberson et al. (2005:400); no error bars are shown because standard error was not reported in that table.
https://doi.org/10.1371/journal.pone.0158725.g007
We sought to determine whether the 2-category model explored above could account for these data. To that end, for each language, we created a version of the 2-category model based on the naming data for that language. Thus, we created an English model in which the two categories were based on empirical naming data for green and blue , a Berinmo model in which the two categories were based on empirical naming data for wor and nol , and a Himba model in which the two categories were based on empirical naming data for dumbu and burou . The black curves in Fig 6 show the probability of assigning a given hue to each of the two native-language categories, according to the category component of a 2-category model fit to each language’s naming data. Given this category information, we simulated color reconstruction from memory for the specific colors considered in the empirical studies [ 9 , 10 ] (the colors in the shaded regions in Fig 6 ). We did so separately for the cases of English, Berinmo, and Himba, in each case fitting a model based on naming data for a given language to discrimination data from speakers of that language. As in Study 1, we fit the model parameter corresponding to the uncertainty of fine-grained perceptual representation to the empirical non-linguistic (here discrimination) data, and we used a single value for this parameter across all three language models. The model results are shown in Fig 7 , beside the empirical data to which they were fit. The models provide a reasonable match to the observed cross-language differences in discrimination. Specifically, the stimulus pairs for which empirical performance is best are those that cross a native-language boundary—and these are stimulus pairs for which the corresponding model response is strongest.
Although not shown in the figure, we also conducted a followup analysis to test whether the quality of these fits was attributable merely to model flexibility, or to a genuine fit between a language’s category system and patterns of discrimination from speakers of that language. We did this by switching which language’s model was fit to which language’s discrimination data. Specifically, we fit the model based on Berinmo naming to the discrimination data from English speakers (and vice versa), and fit the model based on Himba naming to the discrimination data from English speakers (and vice versa), again adjusting the model parameter corresponding to the uncertainty of the fine-grained perceptual representation to the empirical discrimination data. The results are summarized in Table 2 . It can be seen that the discrimination data are fit better by native-language models (that is, models with a category component originally fit to that language’s naming data) than by other-language models (that is, models with a category component originally fit to another language’s naming data). These results suggest that cross-language differences in discrimination may result from category-induced reconstruction bias under uncertainty, guided by native-language categories.
The best value in each row is shown in bold . Data are fit better by native-language models than by other-language models.
https://doi.org/10.1371/journal.pone.0158725.t002
Study 3: Within-category effects
Although many studies of categorical perception focus on pairs of stimuli that cross category boundaries, there is also evidence for category effects within categories. In a 2AFC study of categorical perception of facial expressions, Roberson and colleagues [ 32 ] found the behavioral signature of categorical perception (or more precisely in this case, categorical memory): superior discrimination for cross-category than for within-category pairs of stimuli. But in addition, they found an interesting category effect on within-category pairs, dependent on order of presentation. For each within-category pair they considered, one stimulus of the pair was always closer to the category prototype (the “good exemplar”) than the other (the “poor exemplar”). They found that 2AFC performance on within-category pairs was better when the target was the good exemplar (and the distractor was therefore the poor exemplar) than when the target was the poor exemplar (and the distractor was therefore the good exemplar)—even though the same stimuli were involved in the two cases. Moreover, performance in the former (good exemplar) case did not differ significantly from cross-category performance. Hanley and Roberson [ 36 ] subsequently reanalyzed data from a number of earlier studies that had used 2AFC tasks to explore cross-language differences in color naming and cognition, including those reviewed and modeled in the previous section. Across studies and across domains, including color, they found the same asymmetrical within-category effect originally documented for facial expressions.
This within-category pattern may be naturally explained in category-adjustment terms, as shown in Fig 8 , and as argued by Roberson and colleagues [ 32 ]. The central idea is that because the target is held in memory, it is subject to bias toward the prototype in memory, making discrimination of target from distractor either easier or harder depending on which of the two stimuli is the target. Although this connection with the category adjustment model has been made in the literature in general conceptual terms [ 32 ], followup studies have been theoretically focused elsewhere [ 31 , 36 ], and the idea has not to our knowledge been tested computationally using the specific stimuli and naming patterns involved in the empirical studies. We sought to do so.
The category adjustment model predicts: (top panel, good exemplar) easy within-category discrimination in a 2AFC task when the initially-presented target t is closer to the prototype than the distractor d is; (bottom panel, poor exemplar) difficult within-category discrimination with the same two stimuli when the initially-presented target t is farther from the prototype than the distractor d is. Category is shown as a distribution in blue; stimuli are shown as vertical black lines marked t and d; reconstruction bias patterns are shown as arrows.
https://doi.org/10.1371/journal.pone.0158725.g008
The empirical data in Fig 9 illustrate the within-category effect with published results on color discrimination by speakers of English, Berinmo, and Himba. In attempting to account for these data, we considered again the English, Berinmo, and Himba variants of the 2-category model first used in Study 2, and also retained from that study the parameter value corresponding to the uncertainty of the fine-grained perceptual representation, in the case of native-language models. We simulated reconstruction from memory of the specific colors examined in Study 2. Following the empirical analyses, this time we disaggregated the within-category stimulus pairs into those in which the target was a good exemplar of the category (i.e. the target was closer to the prototype than the distractor was), vs. those in which the target was a poor exemplar of the category (i.e. the target was farther from the prototype than the distractor was). The model results are shown in Fig 9 , and match the empirical data reasonably well, supporting the informal in-principle argument of Fig 8 with a more detailed quantitative analysis.
Across: stimulus pair crosses the native-language boundary; GE: within-category pair, target is the good exemplar; PE: within-category pair, target is the poor exemplar. Empirical data are from Figs 2 (English: 10-second retention interval), 3 (Berinmo), and 4 (Himba) of Hanley and Roberson [ 36 ]. Empirical values show mean proportion correct 2AFC memory judgments, and error bars show standard error. Model values show mean model proportion correct 2AFC memory judgments after simulated reconstruction using native-language categories, range-matched as in Fig 7 . English model compared with English data: 0.00002 MSE; Berinmo model compared with Berinmo data: 0.00055 MSE; Himba model compared with Himba data: 0.00087 MSE.
https://doi.org/10.1371/journal.pone.0158725.g009
Conclusions
We have argued that the debate over the Sapir-Whorf hypothesis may be clarified by viewing that hypothesis in terms of probabilistic inference. To that end, we have presented a probabilistic model of color memory, building on proposals in the literature. The model assumes both a universal color space and language-specific categorical partitionings of that space, and infers the originally perceived color from these two sources of evidence. The structure of this model maps naturally onto a prominent proposal in the literature that has to our knowledge not previously been formalized in these terms. In a classic early study of the effect of language on color cognition, Kay and Kempton [ 7 ] interpret Whorf [ 2 ] as follows:
Whorf […] suggests that he conceives of experience as having two tiers: one, a kind of rock bottom, inescapable seeing-things-as-they-are (or at least as human beings cannot help but see them), and a second, in which [the specific structures of a given language] cause us to classify things in ways that could be otherwise (and are otherwise for speakers of a different language).
Kay and Kempton argue that color cognition involves an interaction between these two tiers. The existence of a universal groundwork for color cognition helps to explain why there are constraints on color naming systems across languages [ 3 – 5 , 37 ]. At the same time, Kay and Kempton acknowledge a role for the language-specific tier in cognition, such that “there do appear to be incursions of linguistic categorization into apparently nonlinguistic processes of thinking” (p. 77). These two tiers map naturally onto the universal and language-specific components of the model we have explored here. This structure offers a straightforward way to think about effects of language on cognition while retaining the idea of a universal foundation underpinning human perception and cognition. Thus, this general approach, and our model as an instance of it, offer a possible resolution of one source of controversy surrounding the Sapir-Whorf hypothesis: taking that hypothesis seriously need not entail a wholesale rejection of important universal components of human cognition.
The approach proposed here also has the potential to resolve another source of controversy surrounding the Sapir-Whorf hypothesis: that some findings taken to support it do not replicate reliably (e.g. in the case of color: [ 15 – 17 ]). Framing the issue in terms of probabilistic inference touches this question by highlighting the theoretically central role of uncertainty , as in models of probabilistic cue integration [ 24 ]. We have seen stronger category-induced bias in color memory under conditions of greater delay and presumably therefore greater uncertainty (Study 1, and [ 20 ]). This suggests that in the inverse case of high certainty about the stimulus, any category effect could in principle be so small as to be empirically undetectable, a possibility that can be pursued by systematically manipulating uncertainty. Thus, the account advanced here casts the Sapir-Whorf hypothesis in formal terms that suggest targeted and quantitative followup tests. A related theoretical advantage of uncertainty is that it highlights an important level of generality: uncertainty could result from memory, as explored here, but it could also result from noise or ambiguity in perception itself, and on the view advanced here, the result should be the same.
The model we have proposed does not cover all aspects of language effects on color cognition. For example, there are documented priming effects [ 31 ] which do not appear to flow as naturally from this account as do the other effects we have explored above. However, the model does bring together disparate bodies of data in a simple framework, and links them to independent principles of probabilistic inference. Future research can usefully probe the generality and the limitations of the ideas we have explored here.
Materials and Methods
Code and data supporting the analyses reported here are available at https://github.com/yangxuch/probwhorfcolor.git .
The perception of stimulus S = s produces a fine-grained memory M , and a categorical code c specifying the category in which s fell. We wish to reconstruct the original stimulus S = s , given M and c .
https://doi.org/10.1371/journal.pone.0158725.g010
Null model.
1-category model.
2-category model.
Fitting models to data.
Participants.
Twenty subjects participated in the experiment, having been recruited at UC Berkeley. All subjects were at least 18 years of age, native English speakers, and reported normal or corrected-to-normal vision, and no colorblindness. All subjects received payment or course credit for participation.
Informed consent was obtained verbally; all subjects read an approved consent form and verbally acknowledged their willingness to participate in the study. Verbal consent was chosen because the primary risk to subjects in this study was for their names to be associated with their response; this approach allowed us to obtain consent and collect data without the need to store subjects’ names in any form. Once subjects acknowledged that they understood the procedures and agreed to participate by stating so to the experimenter, the experimenter recorded their consent by assigning them a subject number, which was anonymously linked to their data. All study procedures, including those involving consent, were overseen and approved by the UC Berkeley Committee for the Protection of Human Subjects.
Stimuli were selected by varying a set of hues centered around the blue - green boundary, holding saturation and lightness constant. Stimuli were defined in Munsell coordinate space, which is widely used in the literature we engage here (e.g. [ 9 , 10 ]). All stimuli were at lightness 6 and saturation 8. Hue varied from 5Y to 10P, in equal hue steps of 2.5. Colors were converted to xyY coordinate space following Table I(6.6.1) of Wyszecki and Stiles (1982) [ 38 ]. The colors were implemented in Matlab in xyY; the correspondence of these coordinate systems in the stimulus set, as well as approximate visualizations of the stimuli, are reported in Table 3 .
All stimuli were presented at lightness 6, saturation 8 in Munsell space.
https://doi.org/10.1371/journal.pone.0158725.t003
- Full range: We collected naming data for green and blue relative to the full range, stimuli 1-27, for a total of 27 stimuli. We fit the category components of our models to naming data over this full range.
- Medium range: We collected bias data for a subset of the full range, namely the medium range, stimuli 5-23, for a total of 19 stimuli. We considered this subset because we were interested in bias induced by the English color terms green and blue , and we had the impression, prior to collecting naming or bias data, that colors outside this medium range had some substantial element of the neighboring categories yellow and purple .
- Focused range: Once we had naming data, we narrowed the range further based on those data, to the focused range, stimuli 5-19, for a total of 15 stimuli. The focused range extends between the (now empirically assessed) prototypes for green and blue , and also includes three of our stimulus hues on either side of these prototypes, yielding a range well-centered relative to those prototypes, as can be seen in the bottom panel of Fig 4 above. We considered this range in our statistical analyses, and in our modeling of bias patterns.
Experimental procedure.
The experiment consisted of four blocks. The first two blocks were reconstruction (bias) tasks: one simultaneous block and one delay block. In the simultaneous block ( Fig 3A ), the subject was shown a stimulus color as a colored square (labeled as “Original” in the figure), and was asked to recreate that color in a second colored square (labeled as “Target” in the figure) as accurately as possible by selecting a hue from a color wheel. The (“Original”) stimulus color remained on screen while the subject selected a response from the color wheel; navigation of the color wheel would change the color of the response (“Target”) square. The stimulus square and response square each covered 4.5 degrees of visual angle, and the color wheel covered 11.1 degrees of visual angle. Target colors were drawn from the medium range of stimuli (stimuli 5—23 of Table 3 ). The color wheel was constructed based on the full range of stimuli (stimuli 1—27 of Table 3 ), supplemented by interpolating 25 points evenly in xyY coordinates between each neighboring pair of the 27 stimuli of the full range, to create a finely discretized continuum from yellow to purple, with 677 possible responses. Each of the 19 target colors of the medium range was presented five times per block in random order, for a total of 95 trials per block. The delay block ( Fig 3B ) was similar to the simultaneous block but with the difference that the stimulus color was shown for 500 milliseconds then disappeared, then a fixation cross was shown for 1000 milliseconds, after which the subject was asked to reconstruct the target color from memory, again using the color wheel to change the color of the response square. The one colored square shown in the final frame of Fig 3B is the response square that changed color under participant control. The order of the simultaneous block and delay block were counterbalanced by subject. Trials were presented with a 500 millisecond inter-trial interval.
Several steps were taken to ensure that responses made on the color wheel during the reconstruction blocks were not influenced by bias towards a particular spatial position. The position of the color wheel was randomly rotated up to 180 degrees from trial to trial. The starting position of the cursor was likewise randomly generated for each new trial. Finally, the extent of the spectrum was jittered one or two stimuli (2.5 or 5 hue steps) from trial to trial, which had the effect of shifting the spectrum slightly in the yellow or the purple direction from trial to trial. This was done to ensure that the blue - green boundary would not fall at a consistent distance from the spectrum endpoints on each trial.
The second two blocks were naming tasks. In each, subjects were shown each of the 27 stimuli of the full range five times in random order, for a total of 135 trials per block. On each trial, subjects were asked to rate how good an example of a given color name each stimulus was. In one block, the color name was green , in the other, the color name was blue ; order of blocks was counterbalanced by subject. To respond, subjects positioned a slider bar with endpoints “Not at all [green/blue]” and “Perfectly [green/blue]” to the desired position matching their judgment of each stimulus, as shown above in Fig 3C . Responses in the naming blocks were self-paced. Naming blocks always followed reconstruction blocks, to ensure that repeated exposure to the color terms green and blue did not bias responses during reconstruction.
The experiment was presented in Matlab version 7.11.0 (R2010b) using Psychtoolbox (version 3) [ 39 – 41 ]. The experiment was conducted in a dark, sound-attenuated booth on an LCD monitor that supported 24-bit color. The monitor had been characterized using a Minolta CS100 colorimeter. A chin rest was used to ensure that each subject viewed the screen from a constant position; when in position, the base of the subject’s chin was situated 30 cm from the screen.
As part of debriefing after testing was complete, each subject was asked to report any strategies they used during the delay block to help them remember the target color. Summaries of each response, as reported by the experimenter, are listed in Table 4 .
When subjects gave specific examples of color terms used as memory aids, they are reported here.
https://doi.org/10.1371/journal.pone.0158725.t004
Color spectrum.
We wished to consider our stimuli along a 1-dimensional spectrum such that distance between two colors on that spectrum approximates the perceptual difference between those colors. To this end, we first converted our stimuli to CIELAB color space. CIELAB is a 3-dimensional color space designed “in an attempt to provide coordinates for colored stimuli so that the distance between the coordinates of any two stimuli is predictive of the perceived color difference between them” (p. 202 of [ 42 ]). The conversion to CIELAB was done according to the equations on pp. 167-168 of Wyszecki and Stiles (1982) [ 38 ], assuming 2 degree observer and D65 illuminant. For each pair of neighboring colors in the set of 677 colors of our color wheel, we measured the distance (Δ E ) betwen these two colors in CIELAB space. We then arranged all colors along a 1-dimensional spectrum that was scaled to length 1, such that the distance between each pair of neighboring colors along that spectrum was proportional to the CIELAB Δ E distance between them. This CIELAB-based 1-dimensional spectrum was used for our analyses in Study 1, and an analogous spectrum for a different set of colors was used for our analyses in Studies 2 and 3.
Statistical analysis.
As a result of the experiment detailed above, we obtained bias data from 20 participants, for each of 19 hues (the medium range), for 5 trials per hue per participant, in each of the simultaneous and delayed conditions. For analysis purposes, we restricted attention to the focused range of stimuli (15 hues), in order to consider a region of the spectrum that is well-centered with respect to green and blue , as we are primarily interested in bias that may be induced by these two categories. We wished to determine whether the magnitude of the bias differed as a function of the simultaneous vs. delayed condition, whether the magnitude of the bias varied as a function of hue, and whether there was an interaction between these two factors. To answer those questions, we conducted a 2 (condition: simultaneous vs. delayed) × 15 (hues) repeated measures analysis of variance (ANOVA), in which the dependent measure was the absolute value of the reproduction bias (reproduced hue minus target hue), averaged across trials for a given participant at a given target hue in a given condition. The ANOVA included an error term to account for across-subject variability. We found a main effect of condition, with greater bias magnitude in the delayed than in the simultaneous condition [ F (1, 19) = 61.61, p < 0.0001], a main effect of hue [ F (14, 266) = 4.565, p < 0.0001], and an interaction of hue and condition [ F (14, 266) = 3.763, p < 0.0001]. All hue calculations were relative to the CIELAB-based spectrum detailed in the preceding section.
We then conducted paired t-tests at each of the target hues, comparing each participant’s bias magnitude for that hue (averaged over trials) in the simultaneous condition vs. the delayed condition. Blue asterisks at the top of Fig 4 mark hues for which the paired t-test returned p < 0.05 when applying Bonferroni corrections for multiple comparisons.
Modeling procedure.
We considered four models in accounting for color reconstruction in English speakers: the null model, a 1-category model for which the category was green , a 1-category model for which the category was blue , and a 2-category model based on both green and blue .
Empirical data.
The empirical data considered for this study were drawn from two sources: the study of 2AFC color discrimination by speakers of Berinmo and English in Experiment 6a of Roberson et al. (2000) [ 9 ], and the study of 2AFC color discrimination by speakers of Himba and English in Experiment 3b of Roberson et al. (2005) [ 10 ]. In both studies, two sets of color stimuli were considered, all at value (lightness) level 5, and chroma (saturation) level 8. Both sets varied in hue by increments of 2.5 Munsell hue steps. The first set of stimuli was centered at the English green - blue boundary (hue 7.5BG), and contained the following seven hues: 10G, 2.5BG, 5BG, 7.5BG, 10BG, 2.5B, 5B. The second set of stimuli was centered at the Berinmo wor - nol boundary (hue 5GY), and contained the following seven hues: 7.5Y, 10Y, 2.5GY, 5GY, 7.5GY, 10GY, 2.5G. Stimuli in the set that crossed an English category boundary all fell within a single category in Berinmo ( nol ) and in Himba ( burou ), and stimuli in the set that crossed a Berinmo category boundary also crossed a Himba category boundary ( dumbu - burou ) but all fell within a single category in English ( green ), according to naming data in Fig 1 of Roberson et al. (2005) [ 10 ]. Based on specifications in the original empirical studies [ 9 , 10 ], we took the pairs of stimuli probed to be those presented in Table 5 .
Any stimulus pair that includes a boundary color is considered to be a cross-category pair. All hues are at value (lightness) level 5, and chroma (saturation) level 8. 1s denotes a 1-step pair; 2s denotes a 2-step pair.
https://doi.org/10.1371/journal.pone.0158725.t005
Based on naming data in Fig 1 of Roberson et al. 2005 [ 10 ], we took the prototypes of the relevant color terms to be:
English green prototype = 10GY
English blue prototype = 10B
Berinmo wor prototype = 5Y
Berinmo nol prototype = 5G
Himba dumbu prototype = 5Y
Himba burou prototype = 10G
Fig 6 above shows a spectrum of hues ranging from the Berinmo wor prototype (5Y) to the English blue prototype (10B) in increments of 2.5 Munsell hue steps, categorized according to each of the three languages we consider here. These Munsell hues were converted to xyY and then to CIELAB as above, and the positions of the hues on the spectrum were adjusted so that the distance between each two neighboring hues in the spectrum is proportional to the CIELAB Δ E distance between them. We use this CIELAB-based spectrum for our analyses below. The two shaded regions on each spectrum in Fig 6 denote the two target sets of stimuli identified above.
The discrimination data we modeled were drawn from Table 11 of Roberson et al. (2000:392) [ 9 ] and Table 6 of Roberson et al. (2005:400) [ 10 ].
We considered three variants of the 2-category model: an English blue - green model, a Berinmo wor - nol model, and a Himba dumbu - burou model. As in Study 1, we fit each model to the data in two steps. For each language’s model, we first fit the category component of that model to naming data from that language. Because color naming differs across these languages, this resulted in three models with different category components. For each model, we then retained and fixed the resulting category parameter settings, and fit the single remaining parameter, corresponding to memory uncertainty, to discrimination data. We detail these two steps below.
The empirical data considered for this study are those of Figs 2 (English green/blue , 10 second delay), 3 (Berinmo wor/nol ), and 4 (Himba dumbu/borou ) of Hanley and Roberson (2011) [ 36 ]. These data were originally published by Roberson and Davidoff (2000) [ 8 ], Roberson et al. (2000) [ 9 ], and Roberson et al. (2005) [ 10 ], respectively. The Berinmo and Himba stimuli and data were the same as in our Study 2, but the English stimuli and data reanalyzed by Hanley and Roberson (2011) [ 36 ] Fig 2 were instead drawn from Table 1 of Roberson and Davidoff (2000) [ 8 ], reproduced here in Table 6 , and used for the English condition of this study. These stimuli for English were at lightness (value) level 4, rather than 5 as for the other two languages. We chose to ignore this difference for modeling purposes.
Any stimulus pair that includes a boundary color is considered to be a cross-category pair. All hues are at value (lightness) level 4, and chroma (saturation) level 8. 1s denotes a 1-step pair; 2s denotes a 2-step pair.
https://doi.org/10.1371/journal.pone.0158725.t006
All modeling procedures were identical to those of Study 2, with the exception that GE (target = good exemplar) and PE (target = poor exemplar) cases were disaggregated, and analyzed separately.
Acknowledgments
We thank Roland Baddeley, Paul Kay, Charles Kemp, Steven Piantadosi, and an anonymous reviewer for their comments.
Author Contributions
Conceived and designed the experiments: EC YX JLA TLG TR. Performed the experiments: EC YX JLA. Analyzed the data: EC YX JLA. Wrote the paper: TR EC YX.
- View Article
- Google Scholar
- 2. Whorf BL. Science and linguistics. In: Carroll JB, editor. Language, Thought, and Reality: Selected Writings of Benjamin Lee Whorf. MIT Press; 1956. p. 207–219.
- 3. Berlin B, Kay P. Basic color terms: Their universality and evolution. University of California Press; 1969.
- 5. Kay P, Berlin B, Maffi L, Merrifield WR, Cook R. The World Color Survey. CSLI Publications; 2009.
- PubMed/NCBI
- 21. Persaud K, Hemmer P. The influence of knowledge and expectations for color on episodic memory. In: Bello P, Guarini M, McShane M, Scassellati B, editors. Proceedings of the 36th Annual Meeting of the Cognitive Science Society. Cognitive Science Society; 2014. p. 1162–1167.
- 22. Yuille AL, Bülthoff HH. Bayesian decision theory and psychophysics. In: Knill DC, Richards W, editors. Perception as Bayesian Inference. Cambridge University Press; 1996. p. 123–162.
- 35. Hemmer P, Persaud K, Kidd C, Piantadosi S. Inferring the Tsimane’s use of color categories from recognition memory. In: Noelle DC, Dale R, Warlaumont AS, Yoshimi J, Matlock T, Jennings CD, et al., editors. Proceedings of the 37th Annual Meeting of the Cognitive Science Society. Cognitive Science Society; 2015. p. 896–901.
- 38. Wyszecki G, Stiles WS. Color science: Concepts and methods, quantitative data and formulae. Wiley; 1982.
- 42. Brainard DH. Color appearance and color difference specification. In: Shevell SK, editor. The science of color: Second edition. Elsevier; 2003. p. 191–216.
- 43. Luce RD. Detection and recognition. In: Luce RD, Bush RR, Galanter E, editors. Handbook of mathematical psychology. Wiley; 1963. p. 103–189.
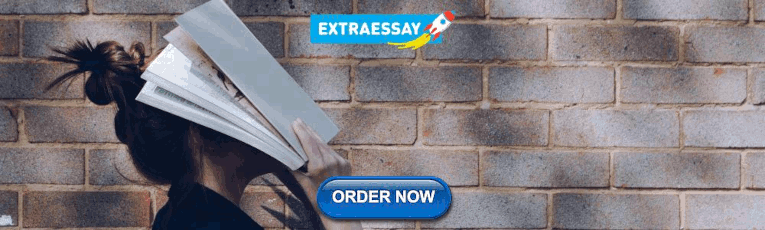
IMAGES
VIDEO
COMMENTS
the hypothesis by linking it to established principles that also explain other phenomena. On this view, effects of language on non-linguistic cognition or perception reflect standard principles of inference under uncertainty. Introduction The Sapir-Whorf hypothesis holds that the semantic categories of one's native language influence
The. Sapir-Whorf hypothesis holds that the seman-tic categories of one's native language influence thought, and that as a result speakers of different lan-guages think differently. This idea has captured the imaginations of many, and has inspired a large litera-ture. However the hypothesis is also controversial, for at least two reasons, one ...
The Sapir-Whorf hypothesis holds that human thought is shaped by language, leading speakers of different languages to think differently. ... On this view, effects of language on nonlinguistic cognition or perception reflect standard principles of inference under uncertainty. WIREs Cogn Sci 2017, 8:e1440. doi: 10.1002/wcs.1440 For further ...
This view may resolve some of the controversy surrounding the Sapir-Whorf hypothesis, and may help to normalize the hypothesis by linking it to established principles that also explain other phenomena. On this view, effects of language on nonlinguistic cognition or perception reflect standard principles of inference under uncertainty.
The Sapir-Whorf hypothesis and inference under uncertainty Terry Regier | Yang Xu Department of Linguistics, Cognitive Science Program, University of California, Berkeley, CA Correspondence Terry Regier, Department of Linguistics, Cognitive Science Program, University of California, Berkeley, CA. Email: [email protected]
The Sapir-Whorf hypothesis and inference under uncertainty. The Sapir-Whorf hypothesis and inference under uncertainty Wiley Interdiscip Rev Cogn Sci. 2018 May;9(3):e1464. doi: 10.1002/wcs.1464. Authors Terry Regier 1 , Yang Xu 1 Affiliation 1 ...
A view of the Sapir-Whorf hypothesis in terms of probabilistic inference is supported, which may resolve some of the controversy surrounding the hypothesis and help to normalize the hypothesis by linking it to established principles that also explain other phenomena. The Sapir-Whorf hypothesis holds that human thought is shaped by language, leading speakers of different languages to think ...
Summarizing the above-mentioned studies, it can be said that the Sapir-Whorf hypothesis about linguistic relativity is unlikely to receive an unambiguous answer like "true" or "false" -a different ...
This view may resolve some of the controversy surrounding the Sapir-Whorf hypothesis, and may help to normalize the hypothesis by linking it to established principles that also explain other phenomena. On this view, effects of language on nonlinguistic cognition or perception reflect standard principles of inference under uncertainty.
Request PDF | On May 1, 2018, Terry Regier and others published The Sapir‐Whorf hypothesis and inference under uncertainty | Find, read and cite all the research you need on ResearchGate
The Sapir-Whorf hypothesis and inference under uncertainty: The Sapir-Whorf hypothesis and inference ... The Sapir-Whorf hypothesis and inference under uncertainty: The Sapir-Whorf hypothesis and inference. NSF-PAR ID: 10035916 Author(s) / Creator(s): Regier, Terry ; Xu, Yang Publisher / Repository: Wiley Blackwell (John Wiley & Sons) Date ...
The Sapir-Whorf hypothesis is captured in the following passage from Sapir:1 Human beings do not live in the objective world alone, nor alone in the world of social activity as ordinarily understood, but are very much at the mercy of the particular language which has become the medium of expression for their society.
This website requires cookies, and the limited processing of your personal data in order to function. By using the site you are agreeing to this as outlined in our privacy notice and cookie policy.
The Sapir-Whorf hypothesis [1,2] holds that our thoughts are shaped by our native language, and that speakers of different languages therefore think about the world in different ways. This proposal has been controversial ... inference under uncertainty, instantiated in a category adjustment model, following Bae et al. [20] and Persaud
Author(s): Regier, Terry; Xu, Yang | Abstract: On page 5, the symbol used in Equation 4 of Box 2 was incorrect; it should be "proportional to" (∝) instead of "equal" (=). The correct presentation of Equations 1-5 is as follows: (Formula presented.) (Formula presented.) (Formula presented.) (Formula presented.) (Formula presented.).
The Sapir-Whorf hypothesis and inference under uncertainty. This article corrects the following:, , Terry Regier, Corresponding Author. Terry Regier [email protected] Department of Linguistics, Cognitive Science Program, University of California, Berkeley, CA. Correspondence. Terry Regier, Department of Linguistics, Cognitive Science Program ...
the hypothesis by linking it to established principles that also explain other phenomena. On this view, effects of language on non-linguistic cognition or perception reflect standard principles of inference under uncertainty. Introduction The Sapir-Whorf hypothesis holds that the semantic categories of one's native language influence
Search worldwide, life-sciences literature Search. Advanced Search Coronavirus articles and preprints Search examples: "breast cancer" Smith J
The Sapir-Whorf hypothesis holds that our thoughts are shaped by our native language, and that speakers of different languages therefore think differently. ... We adopt this stance, and cast color memory as inference under uncertainty, instantiated in a category adjustment model, following Bae et al. and Persaud and Hemmer .
The Sapir-Whorf Hypothesis, also known as linguistic relativity, refers to the idea that the language a person speaks can influence their worldview, thought, and even how they experience and understand the world. While more extreme versions of the hypothesis have largely been discredited, a growing body of research has demonstrated that ...
The Sapir-Whorf hypothesis and inference under uncertainty. Terry Regier, Yang Xu, e1440; First Published: 28 April 2017; ... , Uncertainty as a cognitive control knob, modulating the effect of language on cognition. Abstract; Full text PDF; References; Request permissions; Advanced Reviews. no. False confessions. Saul M. Kassin, e1439; First ...
The Sapir-Whorf hypothesis holds that our thoughts are shaped by our native language, and that speakers of different languages therefore think differently. This hypothesis is controversial in part because it appears to deny the possibility of a universal groundwork for human cognition, and in part because some findings taken to support it have not reliably replicated. We argue that considering ...
The Sapir-Whorf hypothesis holds that our thoughts are shaped by our native language, and that speakers of different languages therefore think differently. This hypothesis is controversial in part because it appears to deny the possibility of a universal groundwork for human cognition, and in part because some findings taken to support it have not reliably replicated. We argue that considering ...